Dynamics of PIP and tidal volume in premature newborns during the first 3 days depending on the mode of MV.
Abstract
Premature newborns are patients who need detailed monitoring of vital processes such as respiration, blood circulation, central and peripheral perfusion, and brain activity. From this point of view, the use of modern methods of visualization of respiratory activity, which can be implemented in the NAVA mode, is a very promising solution to this problem. Our study shows that adequate respiratory support not only contributes to the prevention of ventilator-induced diseases but also reduces the duration of ventilation and the length of stay of patients in the intensive care unit. In addition, this study presents a comparative description of some indicators of ventilation parameters such as peak pressure and tidal volume. We also analyzed the values of gas composition of the venous blood during the first three days. The incidence of bronchopulmonary dysplasia, intraventricular hemorrhages and retinopathy depending on the mode, parameters and duration of ventilation in premature newborns with extremely low birth weight was estimated.
Keywords
- electrical activity of the diaphragm
- premature newborns
- extremely low birth weight
- bronchopulmonary dysplasia
- intraventricular hemorrhages
- retinopathy
1. Introduction
Adequate monitoring of vital functions in preterm infants in the intensive care unit (ICU) is an urgent and quite complicated problem. To get a more complete picture of the severity of child’s condition, the dynamics of the disease and possible outcomes doctors often have to analyze large amounts of information. This implies the study of the maternal history with clarification of somatic or obstetric pathology, interpretation of the results of laboratory and instrumental studies, probing of prescription sheets, which, together with “paper” work, leaves practically no time for direct “communication” with the patient. A substantial problem in the neonatal ICU is lack of objective methods of monitoring the vital functions. Routine measurement of venous blood saturation, heart rate and blood pressure does not adequately assess the severity of the child’s condition and the effectiveness of newly prescribed cardiotonic therapy or the correction of mechanical ventilation (MV) parameters, especially in patients with extremely low body weight (ELBW). Therefore, the doctor has to rely on his own knowledge and experience. In this regard, key points in the progression of the disease may be missed, so it becomes much more difficult to provide quality care to the child. Currently, there is no specialized equipment adapted for this category of patients, which would allow for a combined assessment of the child’s condition, taking into account his neurological deficit, the state of systemic hemodynamics, and the severity of respiratory failure. That is why the issue of using neuromonitoring of the electrical activity of the diaphragm (Edi) of the patient treated in the ICU and requiring MV is extremely promising and interesting.
1.1 Development of the nervous system in a newborn
For a more complete understanding of the principles of action of various aspects of intensive care, whether it is respiratory or cardiotonic support, it is necessary to understand in detail the anatomy and physiology of the respiratory and cardiovascular systems of the premature newborn. The development of the central nervous system (CNS) begins early in utero and continues into the postnatal period. The human brain undergoes slow development from embryonic conception to early adulthood, this being preceded by many events occurring in a strictly limited sequence [1]. The brain develops from the neuroectoderm in the neural plate on the dorsal side of the embryo. Brain stem cells temporarily proliferate with the formation of neurons and glial cells [2]. During pregnancy, the brain undergoes gradual development of various sections, while the central part is formed first and then the peripheral one: proliferative zones (ventricular and subventricular zones), intermediate zone, future white matter (WM), sublamina, cortical plate (future cortex) and marginal zone [3]. Proliferation and migration of neurons predominate in the first trimester of pregnancy, while growth of axons and dendrites occurs mainly in the second and third trimesters. Subsequently, the phenomena of advanced maturation with the mechanisms of synaptogenesis and myelination, neurochemical maturation are observed. At the macroscopic level, intensive brain growth is observed in the last trimester of pregnancy and in the first two years of postnatal period with a significant increase in gray matter volumes and WM [4]. Cortical volume increases from ~10 to ~150 cm3 between 18 and 39 weeks of gestational age and from 200 to 600 cm3 between 1 and 24 months of postnatal age [5]. It is mainly based on an exponential increase in the surface area of the cortex: from ~150 cm2 at 27 weeks and ~2000 cm2 at 1 and 24 months after birth, respectively [6]. This is accompanied by the complication of brain morphology and the formation of convolutions, primary, secondary, and tertiary sulci from 20, 32, and 40 weeks of gestational age [7]. These intense macroscopic changes in volume, surface area and folding are probably visible markers of changes in the microstructure of the cortical plate (the future cortex), which develops by different mechanisms in the preterm and postterm periods. Following the migration of neurons the number of interneuronal connections increases, synaptogenesis actively develops, and the structure of the dendritic tree grows and becomes more complex. In parallel, myelination of intracortical fibers begins, mainly in the early postpartum period. These mechanisms of connection growth, synapse development, and myelination occur at different time periods depending on cortical areas [8]. If we talk about the timing of myelination of specific nerves it should be noted that more primitive, unmyelinated nerve fibers mature first. It is mainly the vagus nerve, which is the first autonomic branch and does not have significant functions before the birth period. [9]. It is followed by the development of the sympathetic department of the nervous system, which develops steadily throughout the prenatal period. [10]. Fetus in utero and preterm infants have natural variability in sympathetic tone, in contrast to parasympathetic tone, which plays an important role in the control of heart rate and blood pressure. On the other hand, the parasympathetic division of the CNS represented by the vagus nerve develops later. The parasympathetic division has little effect until 25–30 weeks of gestation. And at 37–38 weeks there is a sharp increase in the tone of the vagus nerve [11]. Under conditions of intrauterine stress and hypoxia, primitive unmyelinated vagus nerve systems contribute to bradycardia due to imperfect autoregulation [12]. The sucking reflex appears at the 16th week of fetal development, the sucking/swallowing coordination occurs at the 32–34th week of pregnancy, and the interaction of sucking/swallowing with the act of breathing appears only by the end of the 37th week of pregnancy and later. These processes require the involvement of many neuronal signals, including afferent and efferent fibers of the cranial nerves (IV, V, VII, IX, X, XII), nuclei of the inferior medulla oblongata, and motor cortical circuits [13]. Violations in these areas lead to the inability to suck, swallow and breathe. Nervous system symptoms include impaired growth and development of the brain, which are prominent in a variety of emotional, cognitive, motor and social skills. Early assessment and diagnosis of typical signs of developmental anomalies is critical for effective intervention aimed at speedy treatment and minimization of neurological and functional deficits [14]. Otherwise, the disease may progress and result in disability and in some cases death of the child.
In the structure of early neonatal mortality, cerebral injuries occupy a leading place, and, as a rule, they are the result of preterm birth. Brain damage occurs both in the early neonatal and late postnatal periods. Research on neurological diseases in premature babies began in the 1970s. Both experimentally and clinically, a correlation has been found between visualization of cerebral injury and functional outcomes. Since then, much more information has been obtained on the pathophysiology of early brain injury [15]. The most significant acquired brain diseases in preterm infants are periventricular hemorrhages (PVH) and diffuse white matter injury (WMI). The diagnosis of PVH is confirmed by ultrasound examination of the head at the patient’s bedside. For a more complete picture of the nature of brain damage, classifications have been proposed that identify the severity of ischemia and hemorrhage. The most commonly used classifications are Volpe, Papile, Levene, Sarnat, and others. PVH occurs when the fragile vascular network of the germinal matrix (GM) is damaged, at the site where neurons and glial cells are formed. In humans, GM begins to involute around the 28th week of pregnancy and almost completely disappears by the time of birth. The predisposing factors of PVH include the immaturity of the vascular wall of the GM, the dependence of cerebral blood flow on the systemic one, and hemodynamic disorders in premature infants, especially in children with ELBW [16].
In more than 90% of cases, PVH occurs within the first 3 days after birth, when hemodynamic restructuring occurs, and the immature cardiovascular system is maladjusted [17]. Another form of brain damage in preterm infants is white matter damage, with periventricular leukomalacia (PVL) being the main and best known finding. PVL is a focal periventricular necrosis associated with diffuse reactive gliosis and microglial activation in the surrounding white matter [18]. Banker and Larroche were the first to describe cerebral injury in detail and associated it with cardiorespiratory changes and cerebral ischemia [19]. More recently, the neuropathology of WMI was developed by Volpe et al. and received the name “encephalopathy of prematurity”, consisting of a primary destructive disease (acute cell death) and secondary disorders of maturation and trophism, causing insufficiency of differentiation and hypomyelination [20]. It is generally accepted that the main initiating pathogenetic mechanisms of PVL are ischemia and inflammation, and further WMI occur as a result of myelination deficiency and disruption of communication with the CNS pathways. Inflammation leads to microglial activation, free radical attack, and excitotoxicity [21]. Evidence for an association of brain injury caused by hemorrhagic infarction and WMI with poor neurodevelopmental outcomes has been confirmed by neuroimaging and in cohorts around the world. As a rule, cerebral deficiency is manifested by movement disorders, changes in cognition and behavior, deafness and blindness. These manifestations may persist in adult survivors [22].
1.2 Pain and phrenic nerve in premature newborns
Before 1980, there was a long-standing misconception that newborns did not experience pain. However, recent studies have shown that newborns not only experience pain but they are hypersensitive to painful stimuli due to their immature nervous system. The lack of treatment of pain in the neonatal period can lead to negative long-term consequences [23]. Knowledge about pain in newborns has increased dramatically over the past three decades. It is well known that neonates can detect, process and respond to noxious stimuli [24]. Extremely preterm infants are even more hypersensitive to pain due to immature pain suppression mechanisms at birth [25]. Excessive and prolonged painful phenomena in newborns have an adverse effect on all organs and systems, and therefore can be potentially life-threatening [26]. Another problem is determined by inadequate and inconsistent treatment of pain syndrome in newborns. In particular, this applies to the implementation of invasive procedures and care. Despite the fact that neonatology is a progressive science, research on pain in preterm infants is extremely lacking. In 2015, an Oxford research team found evidence that children experience pain in the same way as adults do. MRI scans of ten infants and adults were compared in the presence of a painful stimulus. The results showed that 18 of the 20 brain regions active in adults experiencing pain are also active in newborns. The brain of infants showed the same level of response as in adults, when exposed to a stimulus four times weaker [27]. These results directly contradict the common belief that newborns are unable to perceive pain [28]. At the same time, there were hypotheses confirming this statement. Newborns were thought to be unable to interpret pain due to their inability to create memories. Combined with fears of the side effects of anesthesia, neonatologists performed surgeries such as circumcision without anesthesia until the 1990s [29].
The question of whether term and preterm infants have the necessary anatomy and physiology to sense and respond to pain has been of concern to many researchers. The ability to feel pain occurs at key stages in the development of the nervous system. At the beginning, nociceptors, which perceive a painful stimulus, are involved in the formation of pain reaction. Then they transmit information to the cells surrounding the nociceptors, chemicals are released, which, in turn, act on pain stimuli. In the presence of pain stimuli, the nociceptor transforms the pain signal into an impulse that propagates along the cluster of neurons to the dorsal horns, where sensory information arrives [30]. At this point, the impulse splits: one returns to the original site of pain to trigger a reflex response, the other reaches the thalamus. The thalamus localizes pain and the brain forms information about the nature of pain and how it can be prevented [31]. Each stage of the nociceptive pain pathway corresponds to a specific phase of embryonic development. By the seventh week of pregnancy, nociceptive nerve endings begin to develop around the mouth. And by the 20th week, nociceptive development is completed around the mucous membranes of the body and extremities [32]. However, without any connection to the spine, nociceptor signals are not active and have limited utility. The interaction between nociceptive nerve endings and the posterior horn begins at the 13th week and becomes functional by the 30th week. During this period, the fetus is able to respond to painful stimuli. Cortical pain perception develops after the 24th week of gestation, when the thalamic pathway completes its connection to the dorsal horns. In short, the neonate will be able to localize pain and make reflex movements to try to avoid it after 24 weeks, thus completing the nociceptive pain pathway [33]. Another important component of the pain pathway is the myelin sheath and its role in pain modulation. The myelin sheath acts as an electrical insulator, increasing the speed of signal travel from the peripheral to the central nervous system. Myelination develops after 25 weeks of pregnancy and is completed by the 37th week [34]. Pain modulation is also critical in pain management. Descending signaling pathways exit to the posterior horn, where pain transmission is halted by release of endogenous opioids or activation of inhibitory pathways. However, both of these mechanisms are much more common in adults than in neonates [35]. Thus, in premature infants, the pain threshold is 30–50% lower than in adults, so they have a lower pain tolerance. Subsequent, fatal and recurring painful phenomena have a negative impact on the structures of the brain and the body as a whole. Moreover, they can be life-threatening and have long-term cumulative effects, including altered neurobehavioral development [36].
Another nerve that is of great importance in the intranatal and early postnatal period in newborns is the phrenic nerve. It is the largest nerve of the cervical plexus and is a mixed motor and sensory nerve that originates from the C3–C5 spinal nerves in the neck. The nerve plays a key role in the act of breathing because it provides exclusive motor control of the diaphragm. Any damage at the C4 level or higher can lead to disruption of the connection between the respiratory centers of the brain and the diaphragm. From this point of view, the impact on the phrenic nerve is considered promising in the treatment of cervical injuries and high spinal blocks. Dysfunction of the phrenic nerve plays an important role in the development of respiratory failure in newborns. Because preterm infants, especially those with ELBW, have an underactive peripheral nervous system, they are extremely susceptible to poor phrenic nerve-diaphragm interaction. A similar problem is faced by newborns who have been on MV for a long time. In this case, the main cause is atrophy and secondary weakness of the muscles involved in breathing (including the diaphragm). And, finally, the third category of patients is children with severe neurological deficits, usually of central origin. Thus, all patients are united by one problem—a violation of adequate interaction between the central, peripheral nervous system and the diaphragm. What has been suggested to solve this problem? The first reports of effects on the phrenic nerve appeared in 1976 in patients with tetraplegia [37]. By applying an electrical current to the phrenic nerve, it was possible to cause the diaphragm to contract and thus initiate inspiration. Theoretically, this technique has been known for a long time but the technology that allows stimulation of the phrenic nerve as an alternative to mechanical positive pressure ventilation has existed for only four decades [38]. Establishing phrenic nerve stimulation consists of several steps. The patient’s phrenic nerve and diaphragm must first be assessed to ensure they are interacting and contracting sufficiently to provide adequate airflow. This is done by applying an external current to a transcutaneous nerve in the neck to measure diaphragmatic contraction, and is confirmed by X-ray or ultrasound. If sufficient contraction is demonstrated radioelectrodes are surgically implanted bilaterally and placed directly on the phrenic nerve, and the radio receiver is placed subcutaneously on the chest or abdomen [39, 40]. After that, the technique of endoscopic access to the phrenic nerve appeared. However, both the first and second technologies are accompanied by the invasiveness of the intervention, significant pain syndrome, the need for anesthesia, and the risk of infectious complications. Therefore, these methods are not used in infants. Attempts to treat the phrenic nerve percutaneously in neonates have proved painful and potentially dangerous [41]. From this point of view, the use of NAVA ventilation is not only a painless option for maintaining breathing, but also quite effective in terms of restoring connections between the peripheral nervous system and the diaphragm in extremely immature children. Another key reason for the need for MV in preterm infants is significant lung damage. With the development of neonatal medicine and intensive care, the concepts and methods of treating respiratory diseases in newborns are constantly changing. However, the goal is the same—to minimize lung damage and reduce the incidence of respiratory support related illnesses.
1.3 The negative impact of MV and the development of ventilator-induced diseases in infants
Respiratory distress syndrome (RDS) is a major cause of respiratory failure in preterm infants due to immature lung tissue and surfactant deficiency. This problem does not lose its relevance since the strategies for protecting the lung tissue are not fully understood, although it has been determined that exogenous surfactant therapy, the use of non-invasive ventilation and various ventilation modes to prevent intubation significantly reduce the risks of ventilation-induced lung damage (VILD) and associated diseases [42]. When ventilating the lungs, it is necessary to control the duration of respiratory support, oxygenation targets and peak pressure values. These principles must be followed from the first hours after birth until the moment of extubation. Among conventional ventilation modes, there is strong evidence that volume-triggered ventilation is superior to pressure-limited ventilation. This ventilation reduces the risk of bronchopulmonary dysplasia (BPD), death, and improves long-term outcomes [43]. In addition to BPD, premature infants may suffer from chronic respiratory diseases that prevent them from enduring heavy loads even in adolescence and adulthood [44]. Other complications of ventilation include intracerebral hemorrhages, which often end in cerebral palsy, and retinopathy of prematurity (RP), which ends in blindness [45]. The development of these diseases directly depends on the oxygen concentration in the inhaled mixture, since hyperoxia leads to the development of reactive oxygen species and triggers the process of aseptic inflammation in the corresponding organs [46]. On the other hand, hypoxia increases morbidity and mortality [47]. Thus, saturation level is decisive in clinical practice for correcting the oxygen concentration in the inhaled mixture. This is the ventilation parameter that doctors most often have to change when performing lung ventilation. It should be noted that ventilation is an important aspect of patient care in the intensive care unit especially when it comes to preterm infants. While in past years the main concept of ventilation in newborns was the absence of spontaneous breathing, now it is considered necessary to use modes that promote the preservation of spontaneous breathing and reduce VILD [48]. These include: synchronized intermittent mandatory ventilation (SIMV), assisted controlled ventilation (A/C), and pressure support ventilation (PSV) [49]. In these modes, the start of inspiration is determined by the child’s inspiratory effort, while in A/C mode, all inspiratory attempts that exceed the critical trigger level are supported. In SIMV mode, the ventilator maintains a certain number of breaths set by the doctor, and in the pauses between “hardware” breaths, the patient can breathe on his own. Inspiration occurs when the child’s respiratory activity exceeds the trigger value of flow or pressure, i.e. the patient must create a sufficient change in pressure or flow to induce ventilator support [50]. PSV is a widely used ventilator assist mode, especially during weaning. Although it has proven its value in some clinical conditions, not all of its parameters change depending on the needs of the patient [51]. Using these modes, the doctor arbitrarily sets the values of the peak pressure (PIP) that is supplied to the child, regardless of his needs. Not infrequently, this leads to excess tidal volume, damage to the alveoli and the occurrence of complications. It became possible to reduce the risks of ventilatory complications due to the use of the guaranteed volume (VG) function, which allows you to control the tidal volume [52]. The significance of the effect of tidal volume on the development of complications during MV was proved in the course of a randomized controlled trial, in which two ventilation strategies were evaluated: the first—MV with a high tidal volume (12 ml/kg), the second—with a low tidal volume (6 ml/kg) in adult patients with acute lung injury. The study was terminated prematurely when an interim analysis revealed a significant reduction in mortality and ventilation duration in the low tidal volume group [53]. The use of adequate tidal volume improves stability and reduces lung damage. In addition, limiting rapid changes in arterial carbon dioxide partial pressure by maintaining stable minute ventilation can stabilize cerebral perfusion and reduce brain damage [54]. Despite the constant efforts of doctors to improve the quality of respiratory support using modern ventilation regimens, 40% of children under 28 weeks of age develop BPD [55]. Respiratory therapy strategies for BPD aim to prevent alveolar overexpansion and damage and include avoiding endotracheal intubation when possible, use of non-invasive positive end-expiratory pressure (PEEP) ventilation, and timely administration of surfactant [56]. When a newborn requires endotracheal intubation and MV, it is advisable to use minimal parameters, adhere to permissive hypercapnia, and think about extubation as early as possible [57]. The problem of finding the optimal ventilation mode in children with ELBW and very low birth weight (VLBW) contributed to the introduction of NAVA ventilation into practice. NAVA is a MV mode that initiates inspiration at the same time as the infant attempts to inhale and delivers it in proportion to the Edi, allowing infants to control their own PIP and tidal volume based on the number of inhalations and exhalations. Neural breathing patterns in preterm infants are highly variable, and constant adjustment of NAVA according to inspiratory time and respiratory rate may better adapt to extrauterine life [58]. It is important to remember that whichever ventilation mode or setting you choose, the most important thing is to ensure that the child is adequately ventilated and to minimize lung damage, since the diseases such as BPD, PVH, RP directly depend on the appropriateness and duration of MV. It is these factors that prompted us to use NAVA ventilation as a starting method of respiratory therapy in premature infants, as well as to implement the effectiveness of the proposed therapy by continuously monitoring the Edi.
2. Experience with the use of NAVA ventilation in premature infants in the intensive care unit
2.1 Methods
The study was conducted on the basis of the Irkutsk Regional Perinatal Center. The clinical trial protocol was approved by the ethical committee of this center (decision of the ethical committee No. 10 dated December 27, 2021). The provision of primary resuscitation care to these patients began immediately after birth. A set of measures was carried out in the delivery room, which included the prevention of hypothermia, methods of respiratory stabilization, and surfactant therapy. Respiratory support in the delivery room was performed using the CPAP method with a pressure of 5–6 cm H2O within 10 s followed by an assessment of the heart rate. If spontaneous breathing was not restored within 5 min, ventilation was started with a mask with the following parameters: PIP—20-22 cm H2O, PEEP—5 cm H2O, respiratory rate—60 per minute, fraction of inspired oxygen (FiO2)—21%. In the absence of effective spontaneous breathing, bradycardia of 60–100 beats per minute, as well as in the presence of signs of severe respiratory failure assessed by the Silverman scale tracheal intubation was performed and MV was started through the endotracheal tube. The main indicator of the effectiveness of the proposed respiratory therapy was the increase in heart rate >100 beats/min. Surfactant was administered at a dose of 200 mg/kg in the first 20 min to infants less than 30 weeks gestational age who required tracheal intubation in the delivery room, and to neonates over 30 weeks gestational age who required tracheal intubation in the delivery room with continued FiO2 dependence of more than 40%. Surfactant was administered by the traditional method. Prenatal prevention of RDS was carried out in full in 19 mothers of children of the first group and in 17 mothers of children of the second group. Transportation of patients from the delivery room to the ICU was carried out in a plastic film, in a transport incubator under the control of pulse oximetry by at least two medical workers. In the ICU, all children underwent general clinical, laboratory methods of research, as well as ultrasound examination with Doppler cerebral vessels, echocardiography. Therapeutic measures, which included: infusion and cardiotonic therapy, parenteral nutrition, care features, were performed in accordance with generally accepted clinical protocols.
The work was performed in the design of a cohort, prospective, single-center study. Clinical, instrumental (including monitoring of the Edi), laboratory and statistical research methods were used. The object of the study were premature newborns with ELBW and VLBW at birth with a gestational age of less than 32 weeks.
The aim of the study was to evaluate the role of monitoring the Edi in the complex treatment of premature infants with severe respiratory failure on the background of RDS. Two groups of patients were formed who had common signs: VLBW and ELBW at birth, gestational age 25–32 weeks, need for MV, stable cardiohemodynamics, absence of cerebral lesions according to neurosonography performed on the first day of life, normal gas and electrolyte blood composition before the study, the absence of a generalized infectious process and congenital malformations. Patients of both groups underwent the same complex of diagnostic and treatment measures. In the control group, during respiratory support the SIMV + PS mode with pressure control and flow triggering was used, in the main group the NAVA mode was used, since it is this mode that allows you to continuously monitor the work of the diaphragm. The SIMV + PS mode was used on Avea fans made in the USA and MAQUET Servo-n, Sweden. NAVA ventilation was carried out on MAQUET Servo-n fans with special software, Edi module with cable. The effectiveness of respiratory support was carried out taking into account the assessment of the patient’s respiratory comfort, the presence or absence of asynchrony, the need for sedation, constant monitoring of blood saturation and radiological picture.
To assess gas composition and acid-base composition (ABC) of blood, 0.2 ml of venous blood was taken from the umbilical cord at birth, as well as from the central vein during the first three days. Then pH, partial pressure of carbon dioxide (hvCO2), partial pressure of oxygen (pvO2), base deficiency (BE) and lactate concentration were analyzed during the first three days. In addition, the duration of MV and the duration of treatment in the ICU were analyzed in all patients. The incidence of ventilation associated diseases was also determined.
Statistical data processing was performed using the STATISTICA 10.0 program for Windows. Quantitative data are presented as medians and quartiles (25–75% of the interquartile range). The analysis of the significance of statistical differences in quantitative traits for two independent groups was performed using the Mann-Whitney test, to compare the significance of differences in several traits over time, the Kruskal-Wallis test was used. The analysis of the reliability of statistical differences in qualitative characteristics was carried out using the Pearson x-square test. The value of p < 0.05 was taken as the level of statistical significance.
2.2 Results
2.2.1 Changes in PIP and tidal volume depending on MV
In order to assess the effectiveness and safety of the ventilation modes used in preterm infants, the actual ventilation parameters were studied during the first three days. The tactics of respiratory support in both groups was the same and consisted in using the most sparing parameters to maintain adequate oxygenation and respiratory comfort of the patient. During MV in patients of the first group, we managed to maintain spontaneous breathing, eliminate episodes of asynchrony, and maintain a saturation level of ≥92%. However, to achieve these goals, we were forced to apply a PIP of 16 [15; 17] cm H2O. At the same time, the tidal volume exceeded the physiological norm by several times and amounted to 15.6 [14.6; 16.7] ml. Attempts to reduce PIP in order to restore tidal volume led to the decreased saturation. In order to adequately assess PIP and tidal volume, the same values of PEEP and the FiO2 were used in patients of both groups. PEEP corresponded to 4–5 cm H2O, FiO2 did not exceed 40%. Given that in NAVA mode there is no possibility to arbitrarily set the PIP and its function is performed by the NAVA level which converts the electrical impulse generated by the diaphragm into pressure, our task was to choose the right NAVA level. It allowed us to control the PIP, which changed its value with each breath of the patient. Due to high sensitivity of the trigger, even the smallest attempt to inhale was supported by the ventilator, in proportion to the child’s needs. Thus, the PIP was completely dependent on the patient and amounted to 9 [8; 10] cm H2O (p < 0.01). Despite clear differences with the PIP values obtained during MV in the SIMV+PS mode, in patients with NAVA ventilation we did not observe either a decrease in saturation or changes in the blood gas composition, or a violation of respiratory discomfort. On the contrary, it gave us the opportunity to stabilize the tidal volume and maintain it at the level of 1.5 [1.4; 1.7] ml (p < 0.01). Indicators of PIP and tidal volume in various ventilation modes are shown in Figures 1 and 2.

Figure 1.
Indicators of PIP and tidal volume in a premature baby (body weight 520 g) with respiratory support in the SIMV + PS mode.

Figure 2.
Indicators of PIP and tidal volume in a premature baby (body weight 530 g) with respiratory support in NAVA mode.
Statistically significant differences in PIP and tidal volume values were obtained. The dynamics of PIP and tidal volume in premature newborns during the first three days depending on the ventilation mode is presented in Table 1.
Values | Day | |||||
---|---|---|---|---|---|---|
1 | 2 | 3 | ||||
SIMV + РS | NAVA | SIMV + РS | NAVA | SIMV + РS | NAVA | |
PIP (cm H2O) | 16 [15; 17] | 9 [8; 10] | 17 [16; 18] | 11 [9; 13] | 15 [14; 16] | 10 [9; 11] |
р < 0.01 | ||||||
Volume tidal (ml) | 15.6 [14.6; 16.7] | 1.5 [1.4; 1.7] | 16.0 [15.2; 17.0] | 1.8 [1.5; 2.0] | 15.0 [14.0; 16.0] | 1.7 [1.4; 1.8] |
р < 0.01 |
Table 1.
Note: p—the significance of differences between groups according to the Mann-Whitney test.
So, high PIP and excess tidal volume in patients with respiratory support in SIMV + PS mode are the result of ineffective triggering. In this case, the ventilator does not recognize the child’s breathing attempts, and breaths are delivered automatically.
2.2.2 Dynamics of indicators of gas composition and ABC of blood
The analysis of blood gas composition in patients of the first group revealed hypocapnia. Low levels of pvCO2 in these patients were recorded during the first three days. Despite a significant decrease in pvCO2 in children of the first group, respiratory alkalosis was not observed, which can be explained by compensatory mechanisms. The dynamics of base deficit indicators reflects the compensation of hyperventilation in these patients. The pvCO2 values at birth did not differ significantly and corresponded to normal values. On the first day, children of the control group had hypocapnia. pvCO2 was 32.0 [24.9; 37.8] mm Hg, while in children of the main group pvCO2 values were close to the reference and amounted to 36.0 [32.5; 42.2] mm Hg (p < 0.01). Similar results were obtained on the second and third days. The pvCO2 level in newborns of the control group on the second day was 32.0 [26.7; 38.1] mm Hg, in children of the main group—35.9 [34.2; 40.3] mm Hg (p < 0.01). On the third day pvCO2 values in children receiving MV in the SIMV + PS mode were 33.0 [28.0; 38.3] mm Hg, in children ventilated in the NAVA mode—39.9 [33.7; 43.4] mm Hg (p < 0.02). The dynamics of pvCO2 indicators is shown in Figure 3.
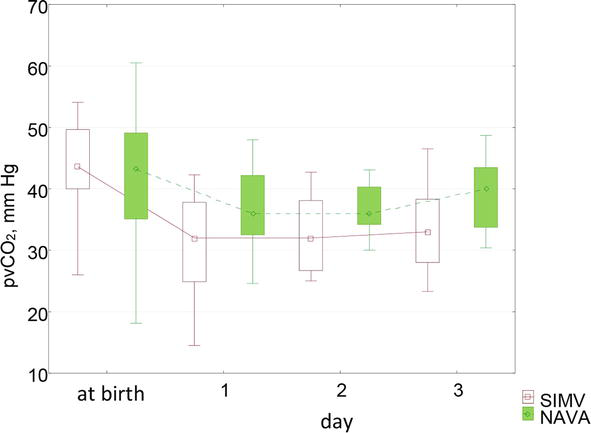
Figure 3.
Dynamics of pvCO2 indicators by day.
The pvO2 values of venous blood at birth in children of the first group were reduced to 22.4 [14.8; 39.4] mm Hg, in children of the second group—to 19.7 [17.8; 25.0] mm Hg, which corresponded to the norm, since the fetus is in a state of physiological hypoxia in utero. Subsequently, there were no significant differences in pvO2 between the groups. On the first day in patients with MV in the SIMV + PS mode, pvO2 values were 37.7 [33.3; 46.8] mm Hg, in patients with NAVA ventilation—43.6 [39.3; 46.9] mm Hg (p = 0.1). On the second and third days, pvO2 was 36.3 [32.9; 42.2] mm Hg and 34.1 [31.0; 40.6] in patients of the control group and 42.4 [37.5; 47.0] mm Hg, 41.2 [36.4; 44.8] mm Hg in patients of the main group (p = 0.2). The daily dynamics of pvO2 indicators is shown in Figure 4.

Figure 4.
Dynamics of pvO2 indicators by day.
The BE indices at birth in children of both groups corresponded to the norm, however, in dynamics in children of the control group, the base deficiency was more pronounced and reached a maximum on the 3rd day. On the first day in children of the control group, the BE values were 5.0 [−7.1; −2.8] mmol/l, in the main group −3.0 [−4.0; −2.0] mmol/l (p < 0.01). On the second day, the level of BE in children of the control group corresponded to −5.7 [−6.8; −4.4] mmol/l, in children of the main group - to-4.0 [−5.2; −2.7] mmol/l (p < 0.01). On the third day it comprised −6.3 [−6.8; −5.2] mmol/l in children of the control group and − 4.7 [−6.0; −3.1] mmol/l in children of the main group (p < 0.02). Changes in the indicators of BE in patients of both groups are shown in Figure 5.
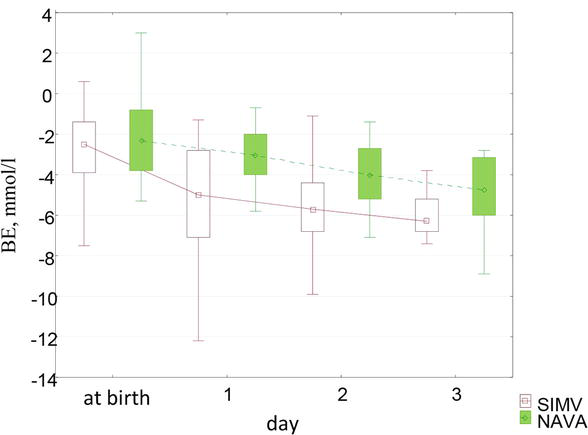
Figure 5.
Dynamics of BE indicators by day.
The level of lactate at birth and during the first three days in children of both groups corresponded to normal values. At birth, its concentration was 2.1 [1.5; 2.6] mmol/l in children of the control group and 2.6 [1.7; 3.7] mmol/l in children of the main group. On the first day in children of the control group, the lactate level was 2.8 [2.5; 4.0] mmol/l compared with 2.5 [2.0; 2.7] mmol/l in children with NAVA ventilation (p = 0.2). On the second day, lactate values in children receiving respiratory support in the SIMV + PS mode corresponded to 2.5 [1.9; 3.5] mmol/l, in children of the main group – to 2.2 [2.1; 2.5] mmol/l (p = 0.26). On the third day, lactate values were 2.6 [2.2; 2.7] mmol/l and 2.1 [1.7; 2.5] mmol/l in children of the first and second groups, respectively (p = 0.11). Thus, the ventilation mode does not affect the level of lactate in the blood. The daily dynamics of lactate concentration is shown in Figure 6.

Figure 6.
Dynamics of lactate by day.
Ph indicators at birth and in dynamics in children of both groups corresponded to the norm and had no statistically significant differences.
2.2.3 Analysis of the incidence of MV associated diseases, the duration of ventilation and the duration of treatment at the intensive care unit
Diseases associated with the damaging effect of MV include PVH of varying severity, BPD and RP. PVH was diagnosed using neurosonography performed on the first, third and seventh days of life. They were verified as thrombi located subependymally (PVH 1st degree), in the lumen of the ventricle without its expansion (PVH 2nd degree), in the lumen of the ventricle with its expansion (PVH 3rd degree), and hemorrhages with a breakthrough into the brain parenchyma (PVH 4th degree). In addition to the direct diagnosis of PVH, some indicators of cerebral hemodynamics were analyzed: blood flow velocity in the anterior, middle and basilar arteries, as well as their resistance indices. PVH was found in 8 patients who underwent MV in the SIMV + PS mode, among them grade 1 hemorrhages were revealed in 5 patients, grade 3 PVH—in 3 patients, grade 2 hemorrhages were not observed. In patients with NAVA ventilation, PVH was diagnosed in 3 cases, with 2 children having grade 1 PVH and 1—grade 2 PVH (p = 0.24). The distribution of patients with PVH depending on the ventilation mode is shown in Figure 7.

Figure 7.
Distribution of patients with PVH depending on the ventilation mode.
The results obtained during the processing of cerebral hemodynamic parameters on the first and third days did not reveal a statistically significant difference either in speed indicators or in the resistance index.
BPD developed in 7 patients of the control group, and there was a clear relationship between the incidence of BPD and the duration of MV. Invasive lung ventilation in these patients was 5 [2; 10] days, and it was noted that the longer the patients underwent MV, the more difficult it was for them to ensure effective spontaneous breathing. In this regard, the duration of treatment in the ICU increased significantly. By the time of transfer to the pathology department for further observation and nursing, the patients developed oxygen dependence. In this regard, they underwent oxygen therapy with the use of binasal cannulas, face masks, or in the form of a background subsidy in the incubator. In the NAVA ventilation group, BPD developed in 1 patient (p < 0.04). The incidence of BPD is shown in Figure 8.

Figure 8.
Incidence of BPD in preterm infants.
All patients included in the study were at risk for developing RP. Primary ophthalmological examination of premature babies born with a gestational age of 25–26 weeks was carried out at 30–31 weeks of postconceptual age; at 27–31 weeks - from 4 weeks of life; at 32 weeks—from 3 weeks of life. The results of the initial examination showed that 5 patients of the control group had RP of various stages, so 3 children had stage 2 RP when the retina in the area of the demarcation line thickened and protruded into the vitreous body. 2 children were diagnosed with stage 3 RP. In the group of children who underwent NAVA ventilation, 3 patients were diagnosed with stage 2 RP (p = 0.35). The outcome of the disease in these patients was as follows: in all patients with stage 2 and in 1 patient with stage 3 RP, the disease regressed, and they did not require surgical treatment. One child had progression of the disease, for which he underwent transpupillary laser coagulation of the retina. The incidence of RP is shown in Figure 9.
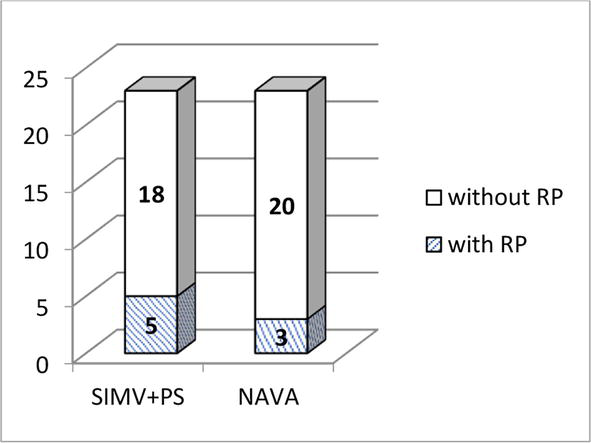
Figure 9.
The incidence of RP in premature newborns.
The duration of respiratory support in patients of the control group was 5 [2; 10] days, in patients of the main group—4 [3; 8] days (p = 0.66), and the duration of stay in the ICU in children of the control group—14 [11; 33] days, in children of the main group—7.0 [6; 15] days (p < 0.01).
3. Conclusion
The performed clinical study showed that continuous monitoring of the Edi not only gives us an idea of the severity of the patient’s condition but also describes in detail his respiratory status and allows you to select ventilation parameters in proportion to the needs of the child, while maintaining a normal blood gas composition. The control of the diaphragm function and the timely adjustment of the ventilator settings allow to maintain spontaneous breathing, ensure good interaction between the child and the ventilator, and reduce the risks of VILD. As a result, the duration of ventilation and the length of stay in the ICU are reduced.
References
- 1.
Dubois J, Alison M. Editors. MRI of the neonatal brain: A review of methodological challenges and neuroscientific advances. Journal of Magnetic Resonance Imaging. 2021; 53 (50):1318-1343. DOI: 10.1002/jmri.27192 - 2.
Lendahl U. Transgenic analysis of central nervous system development and regeneration. Acta Anaesthesiologica Scandinavica Supplementum. 1997; 110 :116-118. DOI: 10.1111/j.1399-6576.1997.tb05524.x - 3.
Gao W, Grewen K, Knickmeyer RC. A review on neuroimaging studies of genetic and environmental influences on early brain development. NeuroImage. 2019; 185 :802-812. DOI: 10.1016/j.neuroimage.2018.04.032 - 4.
Dubois J, Kostovic I, Judas M. Development of structural and functional connectivity. In: Brain Mapping: An Encyclopedic Reference. Vol. 2. Elsevier Cambridge: Academic Press; 2015. pp. 423-437. DOI: 10.1016/B978-0-12-397025-1.00360-2 - 5.
Knickmeyer RC, Gouttard S, Kang C. A structural MRI study of human brain development from birth to 2 years. The Journal of Neuroscience: The Official Journal of the Society for Neuroscience. 2008; 28 (47):12176-12182. DOI: 10.1523/JNEUROSCI.3479-08.2008 - 6.
Lyall AE, Shi F, Geng X. Dynamic development of regional cortical thickness and surface area in early childhood. Cerebral Cortex. 2015; 25 (8):2204-2212. DOI: 10.1093/cercor/bhu027 - 7.
Chi J, Dooling E, Gilles F. Gyral development of the human brain. Annals of Neurology. 1977; 1 (1):86-93. DOI: 101002/ana.410010109 - 8.
Kostović I, Sedmak G, Judaš M. Neural histology and neurogenesis of the human fetal and infant brain. Neuroimage. 2019; 188 :743-773. DOI: 101016/j.neuroimage.2018.12.043 - 9.
Porges SW. In: Schore AN, editor. The Polyvagal Theory. 1st ed. New York: W. W. Norton & Company; 2011. 347 p - 10.
Longin E, Gerstner T. Maturation of the autonomic nervous system: Differences in heart rate variability in premature vs. term infants. Journal of Perinatal Medicine. 2006; 34 :303-308. DOI: 101515/JPM.2006.058 - 11.
Faa G, Manchia M. Fetal programming of neuropsychiatric disorders. Birth Defects Research. Part C, Embryo Today: Reviews. 2016; 108 :207-223. DOI: 101002/bdrc.21139 - 12.
Porges SW, Furman SA. The early development of the autonomic nervous system provides a neural platform for social behavior: A polyvagal perspective. Infant Child Development. 2011; 20 :106-118. DOI: 101002/icd.688 - 13.
Gewolb I, Vice F. Maturational changes in the rhythms, patterning, and coordination of respiration and swallow during feeding in preterm and term infants. Developmental Medicine and Child Neurology. 2006; 48 (7):589-594. DOI: 101017/S001216220600123X - 14.
Friedman H, Soloveichick M. Neuroplasticity in young age: Computer-based early neurodevelopment classifier. In: Neuroplasticity—Insights of Neural Reorganization. Vol. 3. 2017. pp. 47-65. DOI: 105772/intechopen.70894 - 15.
Salmaso N, Jablonska B, editors. Neurobiology of premature brain injury. Nature Neuroscience. 2014; 17 :341-346. DOI: 10.1038/nn.3604 - 16.
Perlman JM. The relationship between systemic hemodynamic perturbations and periventricular-intraventricular hemorrhage—A historical perspective. Seminars Pediatric Neurology. 2009; 16 :191-199. DOI: 10.1016/j.spen.2009.09.006 - 17.
Inder TE, Perlman JM, Volpe JJ. Chapter 24 - preterm intraventricular hemorrhage/posthemorrhagic hydrocephalus. In: Volpe JJ, Inder TE, Darras BT, de Vries LS, du Plessis AJ, Neil JJ, editors. Volpe's Neurology of the Newborn. 6th ed. Elsevier; 2018. pp. 637-698. e21 - 18.
Volpe JJ. Brain injury in premature infants: A complex amalgam of destructive and developmental disturbances. Lancet Neurology. 2009; 8 :110-124. DOI: 101016/S1474-4422(08)70294-1 - 19.
Banker BQ , Larroche JC. Periventricular leukomalacia of infancy. A form of neonatal anoxic encephalopathy. Archives of Neurology. 1962; 7 :386-410. DOI: 101001/archneur.1962.04210050022004 - 20.
Volpe JJ. Confusions in nomenclature: "periventricular leukomalacia" and "white matter injury"-identical, distinct, or overlapping? Pediatric Neurology. 2017; 73 :3-6. DOI: 101016/j.pediatrneurol.2017.05.013 - 21.
Volpe JJ, Kinney HC. The developing oligodendrocyte: Key cellular target in brain injury in the premature infant. International Journal of Developmental Neuroscience. 2011; 29 :423-440. DOI: 101016/j.ijdevneu.2011.02.012 - 22.
Linsell L, Malouf R. Prognostic factors for cerebral palsy and motor impairment in children born very preterm or very low birthweight: A systematic review. Developmental Medicine and Child Neurology. 2016; 58 :554-569. DOI: 101111/dmcn.12972 - 23.
Perry M, Tan Z. Neonatal pain: Perceptions and current practice. Critical Care Nursing Clinics of North America. 2018; 30 (4):549-561. DOI: 101016/j.cnc.2018.07.013 - 24.
Anand K, Hickey P. Pain and its effects in the human neonate and fetus. The New England Journal of Medicine. 1987; 317 (21):1321-1329. DOI: 101056/NEJM198711193172105 - 25.
Fitzgerald M, Beggs S. The neurobiology of pain: Developmental aspects. Neuroscientist. 2001; 7 (3):246-257. DOI: 101177/107385840100700309 - 26.
Grunau R, Holsti L, Haley D. Neonatal procedural pain exposure predicts lower cortisol and behavioral reactivity in preterm infants in the NICU. Pain. 2005; 113 (3):293-300. DOI: 10.1016/j.pain.2004.10.020 - 27.
Goksan S, Hartley C. fMRI reveals neural activity overlap between adult and infant pain. Elife. 2015; 4 :1-13. DOI: 107554/eLife.06356 - 28.
Cong X, Wu J. The impact of cumulative pain/stress on neurobehavioral development of preterm infants in the NICU. Early Human Development. 2017; 108 :9-16. DOI: 101016/j.earlhumdev.2017.03.003 - 29.
Lippmann M, Nelson R. Ligation of patent ductus arteriosus in premature infants. British Journal of Anaesthesia. 1976; 48 (4):365-369. DOI: 101093/bja/48.4.365 - 30.
Brown AG. The dorsal horn of the spinal cord. Quarterly Journal of Experimental Physiology. 1982; 67 (2):193-212. DOI: 101113/expphysiol.1982.sp002630 - 31.
Aziz C, Ahmad A. The role of the thalamus in modulating pain. The Malaysian Journal of Medical Sciences. 2006; 13 (2):11-18 - 32.
Hatfield L. Neonatal pain: What's age got to do with it? Surgical Neurology International. 2014; 13 (5):479-489. DOI: 104103/2152-7806.144630 - 33.
Kostovic I, Rakic P. Developmental history of the transient subplate zone in the visual and somatosensory cortex of the macaque monkey and human brain. Journal of Comparative Neurology. 1990; 297 (3):441-470. DOI: 101002/cne.902970309 - 34.
Hasegawa M, Houdou S. Development of myelination in the human fetal and infant cerebrum: A myelin basic protein immunohistochemical study. Brain Development. 1992; 14 (1):1-6. DOI: 101016/s0387-7604(12)80271-3 - 35.
Fitzgerald M, Walker S. Infant pain management: A developmental neurobiological approach. Nature Clinical Practice. Neurology. 2009; 5 (1):35-50. DOI: 101038/ncpneuro0984 - 36.
Slater R, Fabrizi L. Premature infants display increased noxious-evoked neuronal activity in the brain compared to healthy age-matched term-born infants. Neuroimage. 2010; 52 (2):583-589. DOI: 101016/j.neuroimage.2010.04.253 - 37.
Glenn W, Holcomb W. Central hypoventilation; long-term ventilatory assistance by radiofrequency electrophrenic respiration. Annals of Surgery. 1970; 172 :755-773 - 38.
Glenn W, Holcomb W. Long-term ventilatory support by diaphragm pacing in quadriplegia. Annals of surgery. 1976; 183 :566-577 - 39.
Khong P, Lazzaro A, Mobbs R. Phrenic nerve stimulation: The Australian experience. Journal of Clinical Neuroscience: Official Journal of the Neurological Society of Australia. 2010; 17 :205-208. DOI: 101016/j.jocn.2009.06.012 - 40.
Le Pimpec-Barthes F, Gonzalez-Bermejo J. Intrathoracic phrenic pacing: A 10-year experience in France. Journal of Thoracic and Cardiovascular Surgery. 2011; 142 :378-383. DOI: 101016/j.jtcvs.2011.04.033 - 41.
Similowski T, Fleury B. Cervical magnetic stimulation: A new painless method for bilateral phrenic nerve stimulation in conscious humans. Journal of Applied Physiology. 1989; 67 :1311-1318. DOI: 10.1152/jappl.1989.67.4.1311 - 42.
Zifko U, Hartmann M. Diaphragmatic paresis in newborns due to phrenic nerve injury. Neuropediatrics. 1995; 26 (5):281-284. DOI: 101055/s-2007-979774 - 43.
Schulzke S, Stoecklin B. Update on ventilatory management of extremely preterm infants—A neonatal intensive care unit perspective. Paediatric Anaesthesia. 2022; 32 (2):363-371. DOI: 10.1111/pan.14369 - 44.
Greenough A. Long-term respiratory consequences of premature birth at less than 32 weeks of gestation. Early Human Development. 2013; 89 :25-27. DOI: 101016/j.earlhumdev.2013.07.004 - 45.
Chaves-Samaniego M, Garcia CM. Risk calculator for retinopathy of prematurity requiring treatment. Frontiers in Pediatrics. 2020; 8 :529-639. DOI: 103389/fped.2020.529639 - 46.
Askie L, Henderson-Smart D. Oxygen-saturation targets and outcomes in extremely preterm infants. New England Journal of Medicine. 2003; 349 (10):959-967. DOI: 101056/NEJMoa023080 - 47.
Saugstad O, Aune D. In search of the optimal oxygen saturation for extremely low birth weight infants: A systematic review and meta-analysis. Neonatology. 2011; 100 (1):1-8. DOI: 101159/000322001 - 48.
Gorbachev MO. Effect of neonatal respiratory support strategy in the ICU. Anasteziologiia and Reanimatologiia. 2015; 60 (2):39-43 - 49.
Rocha G. Respiratory care for the ventilated neonate. Canadian Respiratory Journal. 2018; 7 :29-41. DOI: 10.1155/2018/7472964 - 50.
Mellot K. Patient-ventilator dyssynchrony: Clinical significance and implications for practice. Critical Care Medicine. 2009; 29 (6):41-55. DOI: 10.4037/ccn2009612 - 51.
Esteban A. How is mechanical ventilation employed in the intensive care unit? An international utilization review. American Journal Respiratory Critical Care Medicine. 2000; 161 :1450-1458. DOI: 10.1164/ajrccm.161.5.9902018 - 52.
Gorbachev V, Mitkinov O. The use of VTV ventilation mode in premature newborns. Siberian Medical Journal. 2014; 7 :72-75 - 53.
Brower R. Ventilation with lower tidal volumes as compared with traditional tidal volumes for acute lung injury and the acute respiratory distress syndrome. New England Journal of Medicine. 2000; 342 (2):1301-1308. DOI: 10.1056/NEJM200005043421801 - 54.
Wheeler K. Assist control volume guarantee ventilation during surfactant administration. Archives of Disease in Childhood. Fetal and Neonatal Edition. 2009; 94 (5):336-338. DOI: 10.1136/adc.2008.149583 - 55.
Stoll B. Trends in care practices, morbidity, and mortality of extremely preterm neonates 1993-2012. Journal of the American Medical Association. 2015; 314 (10):1039-1051. DOI: 10.1001/jama.2015.10244 - 56.
Jung S. Recent advances in bronchopulmonary dysplasia: Pathophysiology, prevention, and treatment. Lung. 2018; 196 (2):129-138. DOI: 10.1007/s00408-018-0084-z - 57.
Fischer H. Avoiding endotracheal ventilation to prevent bronchopulmonary dysplasia: A meta-analysis. Pediatrics. 2013; 132 (5):1351-1360. DOI: 10.1542/peds.2013-1880 - 58.
Chen I, Chen H. New developments in neonatal respiratory management. Pediatrics and Neonatology. 2022; 63 (4):341-347. DOI: 101016/j.pedneo.2022.02.002