Medical application of nanogels consisting of cholesterol-bearing polysaccharides.
Abstract
Cholesterol-bearing polysaccharides form self-assembled nanogels in water, which are versatile materials suitable for numerous applications in medicine. They are used in cancer vaccines, nasal vaccines, gene delivery, and regenerative medicine. Self-assembled nanogels encapsulate and provide controlled release of various drugs, including proteins (antigens for vaccines and growth hormone for regenerative medicine) or genes (siRNA and plasmid DNA). Moreover, self-assembled nanogel cross-linked macro-sized gels can act as scaffolds to support cell growth and tissue regeneration, making them valuable in tissue engineering and bone repair. Overall, self-assembled nanogels have a variety of medicinal uses and special properties that can improve patient care and progress the medical field.
Keywords
- nanogels
- self-assembly
- cancer vaccine
- nasal vaccine
- gene delivery
- regenerative medicine
1. Introduction
Self-assembled nanogels are created when molecules, including polymers, self-assemble into three-dimensional cross-linked networks, which can entrap therapeutic compounds or medications. The development of self-assembled polymers dates back to the 1980s, when scientists first started looking into the characteristics of self-assembled micelles. Micelles have a core-shell structure using amphiphilic block copolymer molecules with hydrophilic and hydrophobic polymer segments [1]. Following the report of polymer micelles as self-assembled polymer-based nanoparticles, self-assembled nanogels were first reported in 1993 [2], and researchers started looking into the potential of self-assembled nanogels for drug delivery in the 1990s. Compared with alternative drug delivery systems, these materials have a number of advantages, including high stability, biocompatibility, and the capacity to encapsulate both hydrophobic and hydrophilic drugs. Novel varieties of nanogels have been created for various purposes, such as tissue engineering, medication delivery, and diagnostic imaging. In 2007, the International Union for Pure and Applied Chemistry (IUPAC) published a document defining the term “nanogel” [3], and in 2009, a detailed review of nanogels and medical applications was published [4]. Nanogels are regarded as one of the most important formulation classes of nanoparticles. Although various research articles and reviews on nanogels have been published, even in the 2020s, it should be noted that nanogels are different from other polymer-based nanoparticulate formulations, such as polymeric micelles, polymeric nanospheres, and polymersomes. The water content of nanogels (especially hydrogel nanoparticles) should be high because they are nanometer-sized hydro-“GELs.” Cholesterol-bearing polysaccharides contain a high amount of water and are the most studied self-assembled nanogels. This review is focused on cholesterol-bearing polysaccharides, including their history and medical applications (Figure 1).
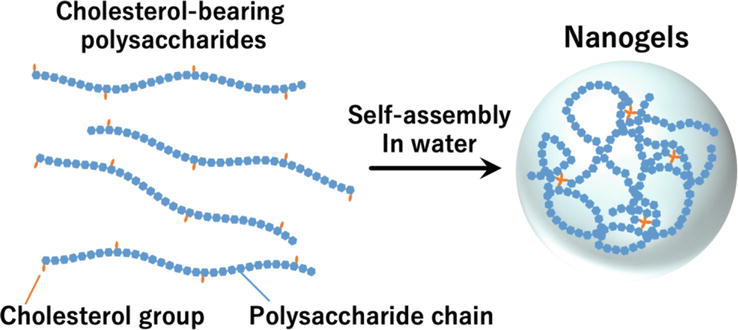
Figure 1.
Self-assembly of cholesterol-bearing polysaccharides.
2. Basic research of self-assembled nanogels
2.1 Pioneer research of self-assembled nanogels
The research related to “supramolecular chemistry,” “self-assembly,” and “host-guest interactions” had been attracted much attentions in the research field of chemistry from 1980s to 2000s [5]. The biomedical applications had been carried out using these self-assembled materials including liposomes [6] and polymeric micelles [1]. In 1993, cholesterol-bearing pullulan (CHP) emerged as one of the first generation of self-aggregating nanogels [2]. Nanogels are nanometer-sized hydrogels. The self-aggregation and complexation behavior of nonionic pullulan derivative CHP-55-1.6, in which cholesterol groups were substituted at 1.6 per 100 glucose units of pullulan (molecular weight of 55,000), in water has been examined. CHP-55-1.6 generates monodispersive nanoparticles, and a single nanoparticle has microdomains comprising several hydrophobic cholesterol cores. The review published in 2009 described that this CHP nanogel is the firstly reported nanogel clearly showing the properties of nanometer-sized hydrogels [4]. In 1997, CHP nanogels with pullulan of various molecular weight pullulan and cholesteryl groups of various degrees of substitution were able to form monodisperse self-assembled nanoparticles in water. As the degree of substitution of the cholesteryl moiety increases, the size of the self-aggregate increases. Cholesteryl moieties are distributed within the particle to create a poly-core structure and to provide non-covalent cross-linking sites for the gels. Therefore, the hydrogel nanoparticle’s overall size and polymer density are readily controlled by substitution degree of cholesteryl group [7].
2.2 Functions of molecular chaperone
Complexation between the CHP nanogel and bovine serum albumin (BSA) or insulin was investigated in 1996 and 1998 [8, 9]. These studies found that the complex of insulin and CHP nanogel is a stable colloid that can resist thermal denaturation and enzymatic degradation, thus maintaining its physiological activity. Subsequently, self-assembled nanogels have been investigated as molecular chaperones. Generally, proteins functioning as molecular chaperones are popular because they can avoid aggregation and misfolding. Owing to their high surface area and capacity to encapsulate proteins, self-assembled nanogels can imitate natural chaperones and function as synthetic molecular chaperones to prevent protein aggregation and misfolding in vitro. By creating a safe environment, nanogels can prevent denaturation of proteins. For example, proteins enclosed in the hydrophobic core of nanogels are protected from hostile environments.
In 1999, CHP nanogels were shown to undergo complexation with carbonic anhydrase B, which is then fully prevented from irreversibly aggregating upon heating. Upon release, the enzyme refolds to take on its natural form, recovering almost all of its original activity. The enzyme’s heat stability is greatly enhanced by the unfolded form that is captured, allowing for efficient refolding [10]. Extensive studies on the use of CHP nanogels in protein refolding were reported in 2003. CHP nanogels act as molecular chaperones and efficiently prevent protein aggregation during the refolding of denatured proteins, such as carbonic anhydrase and citrate synthase. The proteins are released upon dissociation of the gel structure by cyclodextrins (CD), allowing for high enzyme activity. The nanogels trap refolded intermediate proteins and can be used in the renaturation of recombinant proteins from the serine protease family [11]. The dynamic properties of CHP-CD complexes, made of CHP and CD, have been investigated using various types of CDs. CHP nanogels self-regulate protein attachment and dissociation depending on the concentration of CD [12]. Another study examined the effect of CHP nanogels on the colloidal and thermal stability of lipase. The results showed that CHP nanogels significantly increase the enzyme activity and thwart the denaturation and aggregation of the lipase upon heating [13]. In another study, CHP nanogels were used to capture partially or completely unfolded green fluorescent protein. After complexation with methyl-beta-cyclodextrin, CHP nanogels separate to produce dissociated CHP, enabling the release and folding of green fluorescent protein. The folding kinetics are similar to that of spontaneous folding [14]. In a 2011 study, artificial chaperones based on CHP nanogels were used to enhance the folding efficiency of rhodanese and various water-soluble proteins generated in cell-free systems. In the presence of cyclodextrin, proteins fold correctly to produce enzymatically active proteins because the nanogel inhibits protein aggregation [15].
Medical applications have been explored. For example, in 2006, CHP nanogels were used to bind to partially unfolded proteins to prevent protein aggregation. CHP nanogels have been used as artificial chaperones to prevent the development of amyloid beta-protein (Ab) fibrils, which are thought to represent a crucial stage of Alzheimer’s disease. These fibrils contain up to 6–8 Ab molecules, and amine-modified CHP (described below) has been shown to inhibit Ab aggregation [16].
2.3 Varieties of self-assembled nanogels
There are multiple types of self-assembled nanogels. The broad class of self-assembled nanogels can be created by using a wide range of building elements [17]. Research conducted in 2009 discovered that cholesterol modified with a highly branched cyclic dextrin (CH-CDex) can spontaneously form stable, monodisperse nanogels in water. These nanogels exhibit exceptional colloidal stability by capturing insulin. CH-CDex nanogels prevent insulin from dissociating for over 30 days, and in the presence of BSA, insulin is gradually released. A nanogel–protein complex was found to be more stable than the lone protein owing to its highly branched CDex structure [18]. In another study, a monodisperse, spherical, hyperbranched nanoparticle called enzyme-generated glycogen (ESG) was proven to serve as a synthetic chaperone for protein engineering. ESG has a cholesterol group, which enables the creation of amphiphilic ESG nanoballs that can form complexes with proteins. The cluster nanogels are then broken up by cyclodextrin [19]. In a subsequent study using ESG containing hydrophobic groups, amphiphilic ESG nanogels with a radius of 15 nm were found to inhibit carbonic anhydrase from irreversibly aggregating. Without causing the nanogels to separate, cyclodextrin triggers the release of carbonic anhydrase in its active state [20]. Anionic nanogels based on hyaluronic acid (HA) and containing cholesterol groups have been developed for protein delivery. These nanogels can bind to different kinds of proteins without denaturing them, and they can associate with salt to form an injectable hydrogel. According to one study, the level of recombinant human growth hormone (rhGH) in plasma can be preserved for a whole week through the in situ gel formulation of rhGH with HA nanogel, which is a useful way to create sustained protein release systems [21]. In a recent study, HA nanogels were used as molecular chaperones of antibodies to prevent heat denaturation, revealing that HA nanogels can encapsulate a high amount of antibodies and increase the activities of antibodies [22]. In 2017, cholesterol-bearing xyloglucan (CHXG) nanogel was created by incorporating multibranched polysaccharide with xylose and galactose side chains into CHXG nanogel. This nanogel is selectively internalized by hepatocytes via their cell-surface galactose receptors [23].
Recently, several biomedical applications have been reported using hybrid materials of CHP nanogels and inorganic materials, which showed the magnetic responses and named as magnetic nanogel chaperone. Caspase-3 (protease inducing apoptosis) was directly delivered to the target HeLa cells by the magnetic nanogel chaperone after they were guided there by a magnetic field [24]. Subsequent study indicated that saporin (anticancer proteins) can be magnetically steered using magnetic nanogel chaperone in vivo and lowered the tumor’s size in an oral cancer model [25].
3. Cancer immunotherapy
Owing to their effectiveness in delivering antigens to immune cells and in triggering immunological responses, CHP nanogels have attracted interest in the fields of cancer vaccines and cancer immunotherapy [26]. In early studies, CHP nanogels were used to encapsulate stable, long-lasting antigens and thus create cancer vaccines. These vaccines can be made to induce certain cytotoxic T lymphocyte (CTL) responses against cancer cells. Recently, CHP nanogels have been used to deliver antigens to tumors directly and to stimulate immunological responses, with checkpoint inhibitors, against cancer cells. Self-assembled nanogel-based immunotherapeutic drug delivery against cancers is reviewed in this section.
In 1998, a novel hydrophobized polysaccharide nanoparticle formula, (cholesterol-bearing pullulan (CHP) and mannan (CHM) encapsulating human epidermal growth factor receptor 2 (CHP-HER2 and CHM-HER2)) was created to transport an oncogene HER2-containing epitope peptide to the major histocompatibility complex (MHC) class I pathway, generating CTLs and boosting humoral immunity against cancer. In mice, the CHP-HER2 and CHM-HER2 complexes successfully triggered total tumor rejection, in which cluster of differentiation 8 (CD8)-positive T cells were crucial in the effector phase of in vivo tumor rejection. These findings suggest that self-assembled nanogel vaccination is useful for both cancer treatment and prevention [27]. Research further showed that peptides HER2p63-71 and HER2p780-788 (an epitope peptide to the MHC class I pathway) encapsulated in CHP nanogels activated CD8-positive CTLs to act against HER2-positive malignancies, resulting in the total rejection of tumors in mice. This supports the possibility of using researched vaccines for treating and preventing cancer [28]. Subsequently, the use of human dendritic cells in immunotherapy was researched with complexes of CHP nanogels and target proteins (a shortened HER2 protein). These complexes were taken up by human dendritic cells, which then processed the complexes to present the HER2p63 peptide, activating and enhancing CD8-positive T cells with the used peptide’s specificity [29]. In 2006, the results of a human clinical trial using CHP-HER2 vaccination were reported. All processes were carried out under good manufacturing practice (GMP) grade condition. Five patients who received the immunization showed HER2-specific CD8 and CD4-positive T cell immunological responses, indicating that the vaccine was both safe and efficient in eliciting an immune response against HER2-expressing tumors [30]. In a 2007 study, nine patients who received the CHP-NY-ESO-1 complex vaccine showed a rise in the responses of CD8 and CD4-positive T cells, as well as two dominant NY-ESO-1 regions. Local redness at the injection site was shown in all patients, while these reactions disappeared within three days. The safety of subcutaneous administration of CHP nanogel was confirmed [31]. In a research in which patients with malignancies expressing HER were subcutaneously administered vaccines, the CHP-HER2 vaccine induced CD8- and CD4-positive T cell immune responses specific for 146HER2. Moreover, fourteen individuals with baseline negative HER2-specific IgG antibodies had raised 146HER2 antigens. In patients who received the lone CHP-HER2 vaccine, the antibodies were not reactive until the third to sixth immunization. In contrast, in patients who received CHP-HER2 with the granulocyte-macrophage colony-stimulating factor vaccine, antibodies were detectable after the second or third immunization and peaked after the third or fourth round [32]. A 2014 basic study on mice suggested that targeting medullary macrophages is an efficient strategy to induce immunological responses with CHP nanogel-based vaccinations. CHP nanogels were used to encapsulate a synthetic long peptide antigen, which efficiently reached the draining lymph node. Furthermore, long peptides were detected in medullary macrophages but were not detected in other macrophages or dendritic cells. These vaccines significantly inhibited in vivo tumor growth in both prophylactic and therapeutic settings [33]. Researchers also investigated whether a cholesterol-bearing cluster dextrin and CHP nanogels could activate CTLs and thus the T helper 1 (Th1) pathway. In comparison to lone antigen, both self-assembled nanogel vaccines activated CTLs to a greater extent and stimulated the generation of IgG2a antibodies. The distribution of antigens in the draining lymph nodes delivered by nanogels was evaluated, revealing productive interactions with particular groups of cross-presenting dendritic cells [34]. To improve interactions with antigen-presenting cells in the lymphatic system, CHP nanogels were modified to exhibit a net anionic charge by carboxyl group substitution. These nanogels showed effective antigen presentation and significantly increased adaptive immunity [35]. The possibility of combining the CHP nanogel vaccine with an immune checkpoint inhibitor was investigated. An antigen-loaded CHP nanogel with an anti-PD-1 antibody increased the effectiveness of cancer treatments [36]. The above vaccines based on self-assembled nanogels were all administered by subcutaneous injection. In 2019, an antigen with CHP nanogels were systemically administered by intravenous injection to investigate immune resistance in solid tumor models. The results revealed that CD11b and F4/80-expressing tumor-associated macrophages (TAMs) were particularly important for antigen presentation and immune resistance. By intravenously injecting a delivery system targeting TAMs, namely, antigen-loaded CHP nanogels and a Toll-like receptor agonist, antigen-presenting activity in the cancer microenvironment was boosted, and silence tumors were transformed to tumors sensitive to adaptive tumor-specific CTLs [37].
4. Cationic nanogels and its applications (nasal vaccine and gene delivery)
As shown above, early development of self-assembled nanogels for medical applications mainly used CHP nanogels, which have electrically neutral surface charges. Since 2005, cationic group-modified self-assembled nanogels have been prepared for various applications, including intracellular drug delivery, nasal administration, and gene delivery.
4.1 Cationic nanogels
In 2005, monodisperse hybrid nanoparticles were created using quantum dots (QDs) and CHP nanogels modified with amino groups (CHPNH2) to provide novel carriers for intracellular labeling. Compared with traditional carriers, namely, cationic liposomes, CHPNH2-QD complexes demonstrated an improved efficiency for the absorption by multiple human cells [38]. Subsequently, the delivery of intracellular proteins via a CHPNH2 nanogel system was studied. Other traditional cationic drug carriers (cationic liposomes and protein transduction domain-based carriers) were less effective at internalizing HeLa cells in the presence of serum than CHPNH2 nanogels [39]. In another study, CHPNH2 nanogels were shown to provide effective delivery of proteins to myeloma cells and primary CD4-positive T lymphocytes. These cells are often resistant to protein transduction domain-mediated delivery because of inadequate heparan sulfate expression [40].
A different kind of cationic nanogel, namely, cholesterol-bearing cycloamylose modified with spermine (CH-CA-spe), was created for gene delivery. The spermine component enables the CH-CA-spe nanogel to carry genes. Moreover, the polyamine interacts with DNA or RNA molecules through electrostatic interactions to encourage condensation and prevent degradation.
4.2 Nasal vaccines
Owing to their special qualities, including positive charge, size, and stability, cationic nanogels have attracted considerable attention as prospective vaccine delivery systems for nasal administration. Compared with other administration routes, the nasal route has a number of benefits, including the capacity to trigger mucosal and systemic immune reactions, as well as being noninvasive and simple to use. In the nasal mucosa, cationic nanogels can effectively attach to and distribute vaccination antigens for uptake by dendritic cells and other antigen-presenting cells. The positive charge of cationic nanogels interacts with the negative charge of the mucosal surface, which improves the adherence and penetration of cationic nanogels into the nasal epithelium.
In a 2010 research, to evaluate the potential of cationic CHP (cCHP) nanogels for the nasal vaccine delivery system, a nontoxic fragment of
4.3 Gene delivery
A specific kind of cationic nanogel, namely, cholesterol-bearing cycloamylose modified with spermine (CH-CA-spe), was created for gene delivery. The spermine component enables the CH-CA-spe nanogel to carry genes. Moreover, the polyamine interacts with DNA or RNA molecules through electrostatic interactions to encourage condensation and prevent degradation. In the first study, the abilities of CH-CA-spe nanogel and CA-spe to deliver siRNA were investigated. The CH-CA-spe nanogel showed higher gene silencing effect than CA-spe [48]. In 2014, a CH-CA-spe nanogel was tested as a vehicle for siRNA targeting vascular endothelial growth factor (VEGF) in tumor cells. Renal cell carcinoma (RCC) cells ingested the complex of siRNA and CH-CA-spe nanogel, which effectively knocked down VEGF. Intra-tumor injections of the compound drastically reduced RCC development and neovascularization in mice [49]. In another study, cycloamylose was modified with cholesterol and diethylaminoethane to create the CH-CA-DEAE nanogel, which was used to deliver unmethylated CpG oligodeoxynucleotides (immunostimulators). The CH-CA-DEAE nanogel successfully transported native CpG DNA to macrophage-like cells, causing the release of cytokines. Negative control oligonucleotides (with single mutation sample) with the CH-CA-DEAE nanogel prevented cytokine production, and phosphorothioate-modified CpG with the CH-CA-DEAE nanogel reduced cytokine production. These findings suggest that the CH-CA-DEAE nanogel is a potential nucleic acid adjuvant delivery system for native CpG DNA [50].
5. Nanogel tectonic materials and regenerative medicine
Nanogels can be cross-linked to create novel hydrogels known as nanogel-cross-linked (NanoClik) gels. The chemical-crosslinking points of self-assembled nanogels are first modified, and large-scale hydrogels are then prepared using these nanogels as building units. NanoClik gels can be created with different characteristics, including size, shape, and surface charge, and thus, the physical and mechanical characteristics of the resulting macro-sized hydrogel can be adjusted to suit various applications. Since the 2010s, various types of macro-sized NanoClik gels have been developed, known as nanogel tectonic engineering because nanogels are used as tecton units [51].
5.1 Nanogel-cross-linked gels
During the early stage of development, radical polymerization was used to create NanoClik hydrogels, and methacryloyl group-bearing CHP nanogels were used to provide effective cross-linking for gelation. The immobilized nanogels exhibit excellent chaperone-like activity for the refolding of chemically denatured proteins as well as the ability to capture and release protein [52]. Owing to the host–guest interactions of the cholesteryl group and cyclodextrin, the nanogels efficiently trap and release enzymes, which prevents the aggregation of heat-denatured carbonic anhydrase B [53]. In another research, CHP nanogels were modified with acrylate groups (CHPA) and cross-linked by poly(ethylene glycol) containing a thiol group (PEGSH) through the Michael addition reaction. The cross-linking reaction can be conducted under physiological conditions, such as at 37°C, and in the presence of phosphate-buffered saline. Following subcutaneous injection in mice, CHPA nanogels can provide sustained release of encapsulated interleukin-12 (IL-12) in the plasma [54]. By considering the results of various CHP nanogel modifications and cross-linker evaluations, CHP nanogels modified with acryloyl groups (CHPOA) and multi-armed PEGSH have been mostly used to create NanoClik gels. NanoClik gels containing protein-loaded nanogels can gradually release the nanogels by hydrolysis of CHP nanogels and PEGSH [55, 56]. In 2015, a porous NanoClik gel was created. According to two-photon excitation deep imaging, the pores are linked with widths in the range of several hundred micrometers. Owing to its constituent nanogels, the NanoClik porous gel can capture proteins through hydrophobic interactions, thus functioning as a chaperone. Moreover, in vivo evaluation indicated successful penetration of mouse embryonic fibroblasts into the network of interconnected pores [57]. Another study focused on the development of NanoClik microspheres for the manufacture of injectable sustained-release carriers. Self-assembled nanogels encapsulating proteins have been chemically cross-linked with biodegradable linkers to create NanoClik microspheres, which can release “drug-loaded nanogels” with a controlled drug distribution following sustained release, unlike conventional polymeric microspheres, which release naked drugs upon polymer dissolution [58]. In addition to CHP nanogels, cholesterol-bearing hydroxypropyl cellulose (CH-HPC) has been modified with acryloyl groups to prepare macro-scale NanoClik gels. HPC hydrogels are known as thermoresponsive building blocks because they can reversibly vary in size in water. Macrogels cross-linked with CH-HPC nanogels also exhibit this thermoresponsive activity [59].
5.2 Regenerative medicine
NanoClik gels are appealing prospects for a variety of regenerative medicine applications because they may be modified to have desired physical and mechanical properties as well as the capacity to release bioactive compounds in a controlled manner. Nanogel tectonic materials have properties that enable the gradual, regulated release of bioactive compounds, such as high porosity and water-swelling structure. This can be especially helpful for targeted drug delivery to specific tissue locations, and nanogel tectonic materials have been extensively investigated to build three-dimensional scaffolds that resemble the native tissue’s extracellular matrix or tissue engineering.
In 2007, the effectiveness of a bone anabolic drug (prostaglandin E2, PGE2) delivered using a nanogel-based carrier was demonstrated. PGE2 is a hydrophobic small molecule, the encapsulation of PGE2 was conducted in the presence of ethanol. NanoClik gels consisting of CHPA and PEGSH in mice stimulated the creation of new bones without inducing weight loss. PGE2 alone in low doses could not stimulate the growth of new bone, whereas PGE2-loaded Nanoclik gels improved bone development [60]. This synthetic scaffold encapsulating bone morphogenetic proteins (BMP) activated osteoblasts and promoted bone growth for bone regeneration [61]. In a different study, a selective EP4 receptor agonist (EP4A) and low-dose BMP-2 were combined with this type of NanoClik gel. The combined material successfully activated bone cells and restored the outer and inner cortical plates as well as the bone marrow tissue of the calvarium [62]. In 2012, NanoClik gels consisting of CHPOA and PEGSH were used to deliver growth factors in a regulated manner over an extended period of time for bone tissue engineering. The material’s ability to mend broken bones was assessed using BMP-2 and fibroblast growth factor 18, which indicated successful bone regeneration and osteoprogenitor cell infiltration [63]. As a regenerative therapy for tongue muscle abnormalities caused by surgical excision of tongue cancer, NanoCliP gels provided sustained delayed release of protein over the span of 14 days without an initial burst release. Mouse myoblasts attached to the NanoCliP hydrogel exhibited typical myotube development within the gel and remained viable for up to 7 days. Gel-encapsulated myoblasts transplanted into the tongue abnormality of a mouse model resulted in a notable rise in freshly formed myofibers [64]. In 2018, freeze-dried NanoClik porous (NanoCliP-FD) gel was developed to create three-dimensional scaffolds for bone tissue engineering. Fibroblast cells became directly converted osteoblasts (dOBs) by genetic reprograming. The NanoCliP-FD gel effectively supported fibroblast adhesion and formed a matrix of calcified bone after culturing. Animal studies showed that the NanoCliP-FD gel combined with cells reprogramed to dOBs considerably promoted bone regeneration in artificially produced bone defect lesions. These results suggest that the NanoCliP-FD gel is a promising treatment for bone disorders [65]. Subsequently, osteointegrative characteristics were investigated using the NanoCliP-FD gel as a scaffold for differentiation from mesenchymal cells to osteoblasts. Compared with a commercially available atelocollagen scaffold, the NanoCliP-FD gel, which contained chemically and physically cross-linked nanogels inside a porous gel, promoted the growth of apatite crystallites with an unusual c-plane orientation, reflecting the structure of natural enamel [66]. In 2022, researchers used in situ spectroscopic methods, particularly Raman spectroscopy, to show that mesenchymal stem cells cultivated on the NanoCliP-FD gel scaffold exhibited a greater rate of cartilage development and produced tissue [67].
A NanoClik membrane was created using CHPOA nanogels and PEGSH cross-linkers for wound healing. The NanoClik membrane considerably reduced the wound area and increased neoepithelialization. The NanoClik membrane also made it easier for collagen fibers to grow and assemble [68]. NanoCliP-FD sheets and fibers promoted the mending of significant bone lesions and were easy to transplant for bone regeneration. Compared with a control group of unconverted fibroblasts, dOBs treated with NanoCliP-FD in both sheet and fiber forms produced a much higher concentration of calcium deposits [69]. As another example, NanoClik microspheres (with an average diameter of 14 μm) were used to introduce functional support or spacers into cell spheroids. Mesenchymal stem cells from bone marrow were able to combine with NanoClik microspheres to form hybrid cell spheroids. NanoClik microsphere-based spacers were added without adversely affecting cell survival, showing that the microspheres served as a biocompatible scaffold for cell growth. Under culture conditions, the microspheres remained stable for two weeks. The capacity of the hybrid cell spheroids to be scaled up to the millimeter scale was also demonstrated, raising the possibility that they could be transplanted (Table 1) [70].
Application | Nanogels | Complexed with | References |
---|---|---|---|
Cancer vaccines | CHP | HER-2 protein and peptides | [27, 28, 29, 30, 32] |
CHP | NY-ESO-1 protein | [31] | |
CHP | mERK2, MAGE-A4 long peptide | [33, 37] | |
CHP, CHPCOOH | Ovalbumin | [34, 35, 36] | |
Nasal vaccines | CHPNH2 | BoHc/A protein | [41] |
CHPNH2 | PspA protein | [42, 43, 44, 45] | |
CHPNH2 | ghrelin-PspA fusion protein | [46] | |
CHPNH2 | NTHi surface antigen P6 | [47] | |
Gene delivery | CH-CA-spe | siRNA | [48, 49] |
CH-CA-DEAE | CpG DNA | [50] | |
Regenerative medicine | CHPA | PGE2 | [60] |
CHPA | BMP-2 | [61, 62] | |
CHPOA | BMP-2 | [63] | |
CHPOA | Cells | [64, 65, 66, 67] |
Table 1.
6. Conclusion
As described in this review, self-assembled nanogels consisting of cholesterol-bearing polysaccharides are one of the most well-studied biomaterials, and numerous medical applications have been reported in the past three decades. Although over 60 papers are covered, this review does not include all the medical applications of self-assembled nanogels, which have been used not only by researchers in academia but also by medical doctors and people in companies. Even now, there are not commercially available nanogels, while the practical use of the next generation of self-assembled nanogels is desired. In the near future, we hope to see innovative and optical applications of self-assembled nanogels.
Acknowledgments
We thank Edanz (https://jp.edanz.com/ac) for editing a draft of this manuscript.
Conflict of interest
Y. Tahara received funding from Asahi Kasei Corporation related to the research using hyaluronic acid-based nanogels.
References
- 1.
Kataoka K, Harada A, Nagasaki Y. Block copolymer micelles for drug delivery: Design, characterization and biological significance. Advanced Drug Delivery Reviews. 2001; 47 :113-131. DOI: 10.1016/S0169-409X(00)00124-1 - 2.
Akiyoshi K, Deguchi S, Moriguchi N, Yamaguchi S, Sunamoto J. Self-aggregates of hydrophobized polysaccharides in water. Formation and characteristics of nanoparticles. Macromolecules. 1993; 26 :3062-3068. DOI: 10.1021/ma00064a011 - 3.
Alemán JV, Chadwick AV, He J, Hess M, Horie K, Jones RG, et al. Definitions of terms relating to the structure and processing of sols, gels, networks, and inorganic-organic hybrid materials (IUPAC recommendations 2007). Pure and Applied Chemistry. 2007; 79 :1801-1829. DOI: 10.1351/pac200779101801 - 4.
Kabanov AV, Vinogradov SV. Nanogels as pharmaceutical carriers: Finite networks of infinite capabilities. Angewandte Chemie, International Edition. 2009; 48 :5418-5429. DOI: 10.1002/anie.200900441 - 5.
Menger FM. Supramolecular chemistry and self-assembly. Proceedings of the National Academy of Sciences. 2002; 99 :4818-4822. DOI: 10.1073/pnas.062524299 - 6.
Torchilin VP. Recent advances with liposomes as pharmaceutical carriers. Nature Reviews Drug Discovery. 2005; 4 :145-160. DOI: 10.1038/nrd1632 - 7.
Akiyoshi K, Deguchi S, Tajima H, Nishikawa T, Sunamoto J. Microscopic structure and Thermoresponsiveness of a hydrogel nanoparticle by self-assembly of a Hydrophobized polysaccharide. Macromolecules. 1997; 30 :857-861. DOI: 10.1021/ma960786e - 8.
Akiyoshi K, Nishikawa T, Mitsui Y, Miyata T, Kodama M, Sunamoto J. Self-assembly of polymer amphiphiles: Thermodynamics of complexation between bovine serum albumin and self-aggregate of cholesterol-bearing pullulan. Polymer Journal. 1996; 112 :91-95. DOI: 10.1016/0927-7757(96)03560-1 - 9.
Akiyoshi K, Kobayashi S, Shichibe S, Mix D, Baudys M, Wan Kim S, et al. Self-assembled hydrogel nanoparticle of cholesterol-bearing pullulan as a carrier of protein drugs: Complexation and stabilization of insulin. Journal of Controlled Release. 1998; 54 :313-320. DOI: 10.1016/S0168-3659(98)00017-0 - 10.
Akiyoshi K, Sasaki Y, Sunamoto J. Molecular chaperone-like activity of hydrogel nanoparticles of Hydrophobized pullulan: Thermal stabilization with refolding of carbonic anhydrase B. Bioconjugate Chemistry. 1999; 10 :321-324. DOI: 10.1021/bc9801272 - 11.
Nomura Y, Ikeda M, Yamaguchi N, Aoyama Y, Akiyoshi K. Protein refolding assisted by self-assembled nanogels as novel artificial molecular chaperone. FEBS Letters. 2003; 553 :271-276. DOI: 10.1016/S0014-5793(03)01028-7 - 12.
Nomura Y, Sasaki Y, Takagi M, Narita T, Aoyama Y, Akiyoshi K. Thermoresponsive controlled Association of Protein with a dynamic Nanogel of Hydrophobized polysaccharide and Cyclodextrin: Heat shock protein-like activity of artificial molecular chaperone. Biomacromolecules. 2005; 6 :447-452. DOI: 10.1021/bm049501t - 13.
Sawada S-I, Akiyoshi K. Nano-encapsulation of lipase by self-assembled Nanogels: Induction of high enzyme activity and thermal stabilization. Macromolecular Bioscience. 2010; 10 :353-358. DOI: 10.1002/mabi.200900304 - 14.
Sasaki Y, Nomura Y, Sawada S-I, Akiyoshi K. Polysaccharide nanogel–cyclodextrin system as an artificial chaperone for in vitro protein synthesis of green fluorescent protein. Polymer Journal. 2010; 42 :823-828. DOI: 10.1038/pj.2010.73 - 15.
Sasaki Y, Asayama W, Niwa T, Sawada S-I, Ueda T, Taguchi H, et al. Amphiphilic polysaccharide Nanogels as artificial chaperones in cell-free protein synthesis. Macromolecular Bioscience. 2011; 11 :814-820. DOI: 10.1002/mabi.201000457 - 16.
Ikeda K, Okada T, Sawada S-I, Akiyoshi K, Matsuzaki K. Inhibition of the formation of amyloid β-protein fibrils using biocompatible nanogels as artificial chaperones. FEBS Letters. 2006; 580 :6587-6595. DOI: 10.1016/j.febslet.2006.11.009 - 17.
Sasaki Y, Akiyoshi K. Nanogel engineering for new nanobiomaterials: From chaperoning engineering to biomedical applications. The Chemical Record. 2010; 10 :366-376. DOI: 10.1002/tcr.201000008 - 18.
Ozawa Y, Sawada S-I, Morimoto N, Akiyoshi K. Self-assembled Nanogel of Hydrophobized dendritic dextrin for protein delivery. Macromolecular Bioscience. 2009; 9 :694-701. DOI: 10.1002/mabi.200800288 - 19.
Takahashi H, Sawada S-I, Akiyoshi K. Amphiphilic polysaccharide nanoballs: A new building block for Nanogel biomedical engineering and artificial chaperones. ACS Nano. 2011; 5 :337-345. DOI: 10.1021/nn101447m - 20.
Takeda S, Takahashi H, Sawada S-I, Sasaki Y, Akiyoshi K. Amphiphilic nanogel of enzymatically synthesized glycogen as an artificial molecular chaperone for effective protein refolding. RSC Advances. 2013; 3 :25716-25718. DOI: 10.1039/C3RA44572K - 21.
Nakai T, Hirakura T, Sakurai Y, Shimoboji T, Ishigai M, Akiyoshi K. Injectable hydrogel for sustained protein release by salt-induced Association of Hyaluronic Acid Nanogel. Macromolecular Bioscience. 2012; 12 :475-483. DOI: 10.1002/mabi.201100352 - 22.
Antonia-Nancy H, Iwatsuki Y, Yabuuchi K, Aso S, Katsumata T, Fukumoto K, et al. Self-assembled nanogels based on hyaluronic acid for antibody protection from heat denaturation. Biochemical Engineering Journal. 2023; 196 :108955. DOI: 10.1016/j.bej.2023.108955 - 23.
Sawada S-i. Yukawa H, Takeda S, Sasaki Y, Akiyoshi K: Self-assembled nanogel of cholesterol-bearing xyloglucan as a drug delivery nanocarrier. Journal of Biomaterials Science. Polymer Edition. 2017; 28 :1183-1198. DOI: 10.1080/09205063.2017.1320827 - 24.
Kawasaki R, Sasaki Y, Katagiri K, Mukai S-A, Sawada S-I, Akiyoshi K. Magnetically guided protein transduction by hybrid Nanogel chaperones with iron oxide nanoparticles. Angewandte Chemie, International Edition. 2016; 55 :11377-11381. DOI: 10.1002/anie.201602577 - 25.
Kawasaki R, Sasaki Y, Nishimura T, Katagiri K, Morita K-I, Sekine Y, et al. Magnetically navigated protein transduction In vivo using iron oxide-Nanogel chaperone hybrid. Advanced Healthcare Materials. 2021; 10 :2001988. DOI: 10.1002/adhm.202001988 - 26.
Tahara Y, Akiyoshi K. Current advances in self-assembled nanogel delivery systems for immunotherapy. Advanced Drug Delivery Reviews. 2015; 95 :65-76. DOI: 10.1016/j.addr.2015.10.004 - 27.
Gu X-G, Schmitt M, Hiasa A, Nagata Y, Ikeda H, Sasaki Y, et al. A novel Hydrophobized polysaccharide/Oncoprotein complex vaccine induces in vitro and in vivo cellular and humoral immune responses against HER2-expressing murine Sarcomas1. Cancer Research. 1998; 58 :3385-3390. DOI: none - 28.
Shiku H, Wang L, Ikuta Y, Okugawa T, Schmitt M, Gu X, et al. Development of a cancer vaccine: Peptides, proteins, and DNA. Cancer Chemotherapy and Pharmacology. 2000; 46 :S77-S82. DOI: 10.1007/s002800000179 - 29.
Ikuta Y, Katayama N, Wang L, Okugawa T, Takahashi Y, Schmitt M, et al. Presentation of a major histocompatibility complex class 1–binding peptide by monocyte-derived dendritic cells incorporating hydrophobized polysaccharide–truncated HER2 protein complex: Implications for a polyvalent immuno-cell therapy. Blood. 2002; 99 :3717-3724. DOI: 10.1182/blood.V99.10.3717 - 30.
Kitano S, Kageyama S, Nagata Y, Miyahara Y, Hiasa A, Naota H, et al. HER2-specific T-cell immune responses in patients vaccinated with truncated HER2 protein complexed with Nanogels of cholesteryl pullulan. Clinical Cancer Research. 2006; 12 :7397-7405. DOI: 10.1158/1078-0432.CCR-06-1546 - 31.
Uenaka A, Wada H, Isobe M, Saika T, Tsuji K, Sato E, et al. T cell immunomonitoring and tumor responses in patients immunized with a complex of cholesterol-bearing hydrophobized pullulan (CHP) and NY-ESO-1 protein. Cancer Immunity. 2007; 7 :9. DOI: 10.1158/1424-9634.DCL-9.7.1 - 32.
Kageyama S, Kitano S, Hirayama M, Nagata Y, Imai H, Shiraishi T, et al. Humoral immune responses in patients vaccinated with 1-146 HER2 protein complexed with cholesteryl pullulan nanogel. Cancer Science. 2008; 99 :601-607. DOI: 10.1111/j.1349-7006.2007.00705.x - 33.
Muraoka D, Harada N, Hayashi T, Tahara Y, Momose F, Sawada S-I, et al. Nanogel-based immunologically stealth vaccine targets macrophages in the medulla of lymph node and induces potent antitumor immunity. ACS Nano. 2014; 8 :9209-9218. DOI: 10.1021/nn502975r - 34.
Miura R, Tahara Y, Sawada S-I, Sasaki Y, Akiyoshi K. Structural effects and lymphocyte activation properties of self-assembled polysaccharide nanogels for effective antigen delivery. Scientific Reports. 2018; 8 :16464. DOI: 10.1038/s41598-018-34885-8 - 35.
Miura R, Sawada S-I, Mukai S-A, Sasaki Y, Akiyoshi K. Antigen delivery to antigen-presenting cells for adaptive immune response by self-assembled anionic polysaccharide Nanogel vaccines. Biomacromolecules. 2020; 21 :621-629. DOI: 10.1021/acs.biomac.9b01351 - 36.
Miura R, Sawada S-I, Mukai S-A, Sasaki Y, Akiyoshi K. Synergistic anti-tumor efficacy by combination therapy of a self-assembled nanogel vaccine with an immune checkpoint anti-PD-1 antibody. RSC Advances. 2020; 10 :8074-8079. DOI: 10.1039/C9RA10066K - 37.
Muraoka D, Seo N, Hayashi T, Tahara Y, Fujii K, Tawara I, et al. Antigen delivery targeted to tumor-associated macrophages overcomes tumor immune resistance. The Journal of Clinical Investigation. 2019; 129 :1278-1294. DOI: 10.1172/JCI97642 - 38.
Hasegawa U, Nomura S-IM, Kaul SC, Hirano T, Akiyoshi K. Nanogel-quantum dot hybrid nanoparticles for live cell imaging. BBRC. 2005; 331 :917-921. DOI: 10.1016/j.bbrc.2005.03.228 - 39.
Ayame H, Morimoto N, Akiyoshi K. Self-assembled cationic Nanogels for intracellular protein delivery. Bioconjugate Chemistry. 2008; 19 :882-890. DOI: 10.1021/bc700422s - 40.
Watanabe K, Tsuchiya Y, Kawaguchi Y, Sawada S-I, Ayame H, Akiyoshi K, et al. The use of cationic nanogels to deliver proteins to myeloma cells and primary T lymphocytes that poorly express heparan sulfate. Biomaterials. 2011; 32 :5900-5905. DOI: 10.1016/j.biomaterials.2011.04.058 - 41.
Nochi T, Yuki Y, Takahashi H, Sawada S-I, Mejima M, Kohda T, et al. Nanogel antigenic protein-delivery system for adjuvant-free intranasal vaccines. Nature Materials. 2010; 9 :572-578. DOI: 10.1038/nmat2784 - 42.
Kong Il G, Sato A, Yuki Y, Nochi T, Takahashi H, Sawada S, et al. Nanogel-based PspA intranasal vaccine prevents invasive disease and nasal colonization by Streptococcus pneumoniae. Infection and Immunity. 2013; 81 :1625-1634. DOI: 10.1128/iai.00240-13 - 43.
Fukuyama Y, Yuki Y, Katakai Y, Harada N, Takahashi H, Takeda S, et al. Nanogel-based pneumococcal surface protein a nasal vaccine induces microRNA-associated Th17 cell responses with neutralizing antibodies against Streptococcus pneumoniae in macaques. Mucosal Immunology. 2015; 8 :1144-1153. DOI: 10.1038/mi.2015.5 - 44.
Nakahashi-Ouchida R, Uchida Y, Yuki Y, Katakai Y, Yamanoue T, Ogawa H, et al. A nanogel-based trivalent PspA nasal vaccine protects macaques from intratracheal challenge with pneumococci. Vaccine. 2021; 39 :3353-3364. DOI: 10.1016/j.vaccine.2021.04.069 - 45.
Yuki Y, Uchida Y, Sawada S-I, Nakahashi-Ouchida R, Sugiura K, Mori H, et al. Characterization and specification of a trivalent protein-based pneumococcal vaccine formulation using an adjuvant-free Nanogel nasal delivery system. Molecular Pharmaceutics. 2021; 18 :1582-1592. DOI: 10.1021/acs.molpharmaceut.0c01003 - 46.
Azegami T, Yuki Y, Sawada S, Mejima M, Ishige K, Akiyoshi K, et al. Nanogel-based nasal ghrelin vaccine prevents obesity. Mucosal Immunology. 2017; 10 :1351-1360. DOI: 10.1038/mi.2016.137 - 47.
Nakahashi-Ouchida R, Mori H, Yuki Y, Umemoto S, Hirano T, Uchida Y, et al. Induction of mucosal IgA–mediated protective immunity against Nontypeable haemophilus influenzae infection by a cationic Nanogel–based P6 nasal vaccine. Frontiers in Immunology. 2022; 13 :819859. DOI: 10.3389/fimmu.2022.819859 - 48.
Toita S, Soma Y, Morimoto N, Akiyoshi K. Cycloamylose-based biomaterial: Nanogel of cholesterol-bearing cationic Cycloamylose for siRNA delivery. Chemistry Letters. 2009; 38 :1114-1115. DOI: 10.1246/cl.2009.1114 - 49.
Fujii H, Shin-Ya M, Takeda S, Hashimoto Y, Mukai S-A, Sawada S-I, et al. Cycloamylose-nanogel drug delivery system-mediated intratumor silencing of the vascular endothelial growth factor regulates neovascularization in tumor microenvironment. Cancer Science. 2014; 105 :1616-1625. DOI: 10.1111/cas.12547 - 50.
Tahara Y, Yasuoka J, Sawada S, Sasaki Y, Akiyoshi K. Effective CpG DNA delivery using amphiphilic cycloamylose nanogels. Biomaterials Science. 2015; 3 :256-264. DOI: 10.1039/C4BM00293H - 51.
Hashimoto Y, Mukai S-A, Sasaki Y, Akiyoshi K. Nanogel tectonics for tissue engineering: Protein delivery systems with Nanogel chaperones. Advanced Healthcare Materials. 2018; 7 :1800729. DOI: 10.1002/adhm.201800729 - 52.
Morimoto N, Endo T, Iwasaki Y, Akiyoshi K. Design of Hybrid Hydrogels with self-assembled Nanogels as cross-linkers: Interaction with proteins and chaperone-like activity. Biomacromolecules. 2005; 6 :1829-1834. DOI: 10.1021/bm050156x - 53.
Morimoto N, Endo T, Ohtomi M, Iwasaki Y, Akiyoshi K. Hybrid Nanogels with physical and chemical cross-linking structures as Nanocarriers. Macromolecular Bioscience. 2005; 5 :710-716. DOI: 10.1002/mabi.200500051 - 54.
Hasegawa U, Sawada S-I, Shimizu T, Kishida T, Otsuji E, Mazda O, et al. Raspberry-like assembly of cross-linked nanogels for protein delivery. Journal of Controlled Release. 2009; 140 :312-317. DOI: 10.1016/j.jconrel.2009.06.025 - 55.
Shimoda A, Sawada S-I, Kano A, Maruyama A, Moquin A, Winnik FM, et al. Dual crosslinked hydrogel nanoparticles by nanogel bottom-up method for sustained-release delivery. Colloids and Surfaces, B: Biointerfaces. 2012; 99 :38-44. DOI: 10.1016/j.colsurfb.2011.09.025 - 56.
Shimoda A, Yamamoto Y, Sawada S-I, Akiyoshi K. Biodegradable nanogel-integrated hydrogels for sustained protein delivery. Macromolecular Research. 2012; 20 :266-270. DOI: 10.1007/s13233-012-0054-x - 57.
Hashimoto Y, Mukai S-A, Sawada S-I, Sasaki Y, Akiyoshi K. Nanogel tectonic porous gel loading biologics, nanocarriers, and cells for advanced scaffold. Biomaterials. 2015; 37 :107-115. DOI: 10.1016/j.biomaterials.2014.10.045 - 58.
Tahara Y, Mukai S-A, Sawada S-I, Sasaki Y, Akiyoshi K. Nanocarrier-integrated microspheres: Nanogel tectonic engineering for advanced drug-delivery systems. Advanced Materials. 2015; 27 :5080-5088. DOI: 10.1002/adma.201501557 - 59.
Tahara Y, Sakiyama M, Takeda S, Nishimura T, Mukai S-A, Sawada S-I, et al. Self-assembled Nanogels of cholesterol-bearing Hydroxypropyl cellulose: A Thermoresponsive building block for Nanogel tectonic materials. Langmuir. 2016; 32 :12283-12289. DOI: 10.1021/acs.langmuir.6b02406 - 60.
Kato N, Hasegawa U, Morimoto N, Saita Y, Nakashima K, Ezura Y, et al. Nanogel-based delivery system enhances PGE2 effects on bone formation. Journal of Cellular Biochemistry. 2007; 101 :1063-1070. DOI: 10.1002/jcb.21160 - 61.
Hayashi C, Hasegawa U, Saita Y, Hemmi H, Hayata T, Nakashima K, et al. Osteoblastic bone formation is induced by using nanogel-crosslinking hydrogel as novel scaffold for bone growth factor. Journal of Cellular Physiology. 2009; 220 :1-7. DOI: 10.1002/jcp.21760 - 62.
Kamolratanakul P, Hayata T, Ezura Y, Kawamata A, Hayashi C, Yamamoto Y, et al. Nanogel-based scaffold delivery of prostaglandin E2 receptor–specific agonist in combination with a low dose of growth factor heals critical-size bone defects in mice. Arthritis and Rheumatism. 2011; 63 :1021-1033. DOI: 10.1002/art.30151 - 63.
Fujioka-Kobayashi M, Ota MS, Shimoda A, Nakahama K-I, Akiyoshi K, Miyamoto Y, et al. Cholesteryl group- and acryloyl group-bearing pullulan nanogel to deliver BMP2 and FGF18 for bone tissue engineering. Biomaterials. 2012; 33 :7613-7620. DOI: 10.1016/j.biomaterials.2012.06.075 - 64.
Kinoshita N, Sasaki Y, Marukawa E, Hirose R, Sawada S-I, Harada H, et al. Crosslinked nanogel-based porous hydrogel as a functional scaffold for tongue muscle regeneration. Journal of Biomaterials Science. Polymer Edition. 2020; 31 :1254-1271. DOI: 10.1080/09205063.2020.1744246 - 65.
Sato Y, Yamamoto K, Horiguchi S, Tahara Y, Nakai K, Kotani S-I, et al. Nanogel tectonic porous 3D scaffold for direct reprogramming fibroblasts into osteoblasts and bone regeneration. Scientific Reports. 2018; 8 :15824. DOI: 10.1038/s41598-018-33892-z - 66.
Horiguchi S, Adachi T, Rondinella A, Boschetto F, Marin E, Zhu W, et al. Osteogenic response of mesenchymal progenitor cells to natural polysaccharide nanogel and atelocollagen scaffolds: A spectroscopic study. Materials Science and Engineering: C. 2019; 99 :1325-1340. DOI: 10.1016/j.msec.2019.02.043 - 67.
Adachi T, Miyamoto N, Imamura H, Yamamoto T, Marin E, Zhu W, et al. Three-dimensional culture of cartilage tissue on Nanogel-cross-linked porous freeze-dried gel scaffold for regenerative cartilage therapy: A vibrational spectroscopy evaluation. International Journal of Molecular Sciences. 2022; 23 :8099. DOI: 10.3390/ijms23158099 - 68.
Maeda H, Kobayashi H, Miyahara T, Hashimoto Y, Akiyoshi K, Kasugai S. Effects of a polysaccharide nanogel-crosslinked membrane on wound healing. Journal of Biomedical Materials Research. 2017; 105 :544-550. DOI: 10.1002/jbm.b.33571 - 69.
Nakai K, Yamamoto K, Kishida T, Kotani S-i, Sato Y, Horiguchi S, et al. Osteogenic response to polysaccharide Nanogel sheets of human fibroblasts after conversion into functional osteoblasts by direct phenotypic cell reprogramming. Frontiers in Bioengineering and Biotechnology. 2021; 9 :713932. DOI: 10.3389/fbioe.2021.713932 - 70.
Hayashi S, Sasaki Y, Kubo H, Sawada S-I, Kinoshita N, Marukawa E, et al. Construction of hybrid cell spheroids using cell-sized cross-linked Nanogel microspheres as an artificial extracellular matrix. ACS Applied Bio Materials. 2021; 4 :7848-7855. DOI: 10.1021/acsabm.1c00796