Overview of hydrogelators, CGCs, drug trapping, and release conditions.
Abstract
Low-molecular-weight hydrogels (LMWHs) have garnered widespread focus as versatile soft materials owing to their distinctive characteristics and potential applications. LMWHs are synthesized from small molecules that, upon assembly, form entangled aggregates via different types of noncovalent interactions, such as hydrogen bonding, van der Waals forces, or π-π stacking interactions. LMWHs are characterized by their unique ability to mimic biological systems by effectively absorbing and retaining large quantities of water. Despite their poor mechanical properties, LMWHs are widely used in various medical applications due to their easy preparation, biocompatibility, and low toxicity. Smart LMWHs demonstrate responsiveness to external stimuli, such as light, temperature, enzymes, or pH, rendering them ideally adapted for various controlled drug delivery applications. LMWHs have been extensively employed in different biomedical applications, including drug delivery, tissue engineering and cell culture, wound healing, and biofabrication. In this chapter, we aim to explore the potential of LMWHs as drug-delivery vehicles for a range of medications, focusing on the different synthetic strategies, gelation processes, and drug-loading and releasing mechanisms.
Keywords
- low-molecular-weight hydrogels
- smart hydrogels
- drug delivery
- biomedical applications
- stimuli-responsive
- gelation mechanisms
- noncovalent interactions
1. Introduction
Low-molecular-weight hydrogels (LMWHs) have gained significant attention across various disciplines as an important class of soft materials. These gels are composed of small organic molecules that can self-assemble in water, forming 3D network structures. The self-assembly of LMWHs into entangled networks is typically driven by various noncovalent interactions, such as hydrogen bonding, π-π stacking, or van der Waals forces. The noncovalent interactions between the molecules result in the formation of fibrous structures that become entangled and form hydrogels. LMWHs are known for their high water retention capability, which imparts them with a resemblance to biological tissues. LMWHs have been widely studied in various biomedical research areas due to their biocompatibility, tailored design, cost-effectiveness, and ease of preparation. LMWHs show great potential as a drug delivery platform, as they can encapsulate drugs and release them in a slow and sustained manner. For instance, LMWHs have been used to deliver vascular endothelial growth factor small interfering RNA (VEGF-siRNA) into human cells [1]. LMWHs derived from vitamin B have been utilized in the delivery of siRNA into cancer cell lines with high selectivity [2]. LMWHs have also been exploited for the delivery of proteins [3, 4].
In addition to their use in drug delivery, LMWHs have exhibited significant potential in several medicinal applications. LMWHs with inherent antibacterial properties have been widely explored [5, 6, 7]. Adams
This chapter aimed to provide a comprehensive understanding of the design, synthesis, and applications of LMWHs in drug delivery. We discussed the various synthetic methods utilized for the fabrication of LMWHs and highlighted the challenges of using LMWHs as drug carriers along with possible solutions. In addition, we reviewed the different drug cargos that have been utilized in drug delivery purposes incorporating LMWHs. These drugs included anticancer, anti-inflammatory, antibacterial agents, and vitamins, to name a few. The versatility and potential of LMWHs as drug-delivery vehicles in various therapeutic areas were emphasized. Furthermore, we provided insights into the mechanism of drug release from LMWHs. We also discussed the influence of the chemical structure of LMWHs on drug delivery efficiency, highlighting in particular, the importance of their functionalization.
2. Exploring the gelation process of LMWHs
LMWHs can form by self-assembly of small molecular weight molecules into 3D network structures capable of immobilizing water. The gelation process can be optimized by testing different parameters and observing their influences on gelation. These parameters include, but are not limited to, the concentration of the gelator, the temperature of gelation, the pH of the solution, and the addition of an external agent to promote gelation. Optimizing these parameters can aid in fine-tuning the conditions required for the organic molecules to dissolve freely in water upon heating and form a clear solution. Upon cooling, the solution can turn into a gel, which can be studied to explore the factors that promote and reinforce the gelation. The inclusion of functional motifs, or groups capable of establishing hydrogen bonding or π-π stacking, into the structural backbone of the gelator is also crucial for promoting the gelation process [23]. Therefore, by testing and optimizing these parameters, the gelation process of LMWHs can be improved and tailored for specific applications.
3. LMWHs in drug delivery: Opportunities and challenges
LMWHs possess several advantages that make them excellent candidates for various drug delivery applications, such as biocompatibility, ease of preparation, structural customization, stimuli-responsiveness, versatility, and controlled drug release. When drugs are loaded into the hydrogel matrix, they gain extra stability against degradation, oxidation, and other adverse effects, leading to a longer shelf life. Additionally, controlled drug release is a crucial benefit of using drug-loaded LMWHs, as it reduces dosing frequency. LMWHs can be tailored to deliver drugs to specific areas, which is especially useful in the case of anticancer medications, as it helps avoid harming healthy cells. Injecting anti-inflammatory and analgesic drugs into sites of injury or inflammation can also provide prolonged pain relief. Moreover, the high water content of LMWHs makes them ideal for encapsulating various drugs. The synthesis of LMWHs is often straightforward and cost-effective when compared to other drug delivery systems.
Despite their intriguing properties, using LMWHs for drug delivery may have some critical limitations. For example, some LMWHs may have weak mechanical strength, a limited capacity for drug loading, and insufficient drug release, which could influence their therapeutic effectiveness. In addition, some LMWHs may not be suitable for encapsulating drugs with poor solubility or achieving targeted drug delivery. Furthermore, certain LMWHs may have low stability for in
To improve the mechanical strength of hydrogels co-assembly can be utilized. For instance, acylhydrazone-based hydrogels incorporating bipyridinium units have been found to form highly stable hydrogels through donor-acceptor charge transfer interactions with electron-rich naphthyl moieties [24, 25]. The addition of metal ions can also enhance the mechanical properties and thermal stability of LMWHs [26]. The design of LMWHs can greatly impact their loading capacity, pH and temperature stability, targeted delivery ability,
Drug loading into the hydrogel matrix can be accomplished using physical entrapment or chemical modification of the structure of the hydrogelator, with the former relying on noncovalent interactions and the latter utilizing covalent binding. Nevertheless, both methods have notable drawbacks. Certain drugs may not establish strong enough interactions with the hydrogelator, which can result in rapid drug release, while others may form overly strong interactions, leading to very slow drug release. Chemical modification of the gelators to integrate drugs into their structural framework can lead to a decrease in the drug’s effectiveness after its release from the hydrogel structure. For example, the incorporation of ciprofloxacin into the structure of a peptide gelator resulted in a reduction of its antibacterial activity [27]. Therefore, it is highly important to further investigate the process of linking drugs into the backbone structure of the gelators as a drug delivery approach. Additionally, it is crucial to ensure controlled and sustained drug release, especially for physically entrapped drugs, in order to maintain the drugs’ efficacy and optimize therapeutic outcomes.
Another important challenge facing drug delivery using LMWHs is the limited drug-loading capacity. This obstacle can be overcome by modifying the hydrogel structure, such as by incorporating functional motifs or groups that reinforce the drug’s affinity for the hydrogelator. Moreover, optimizing drug concentration can also impact the drug-loading capacity. The drug loading can also be improved by increasing the porosity of the gelator. In addition, considering the pre-loading or post-loading of drugs into the hydrogel matrix is a critical factor in achieving a high loading capacity. These strategies are essential to developing effective drug delivery systems with higher drug loading capacities.
4. Applications of LMWHs in drug delivery
Hydrogel
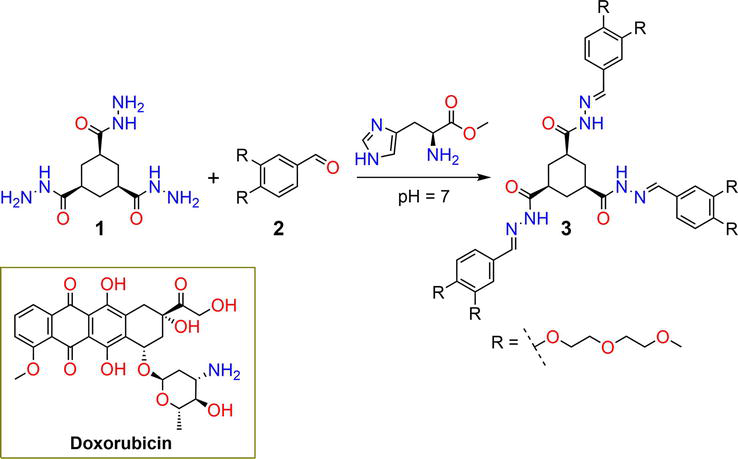
Figure 1.
Synthetic pathway of hydrogelator
The biocompatibility of the gel was evaluated
Banerji
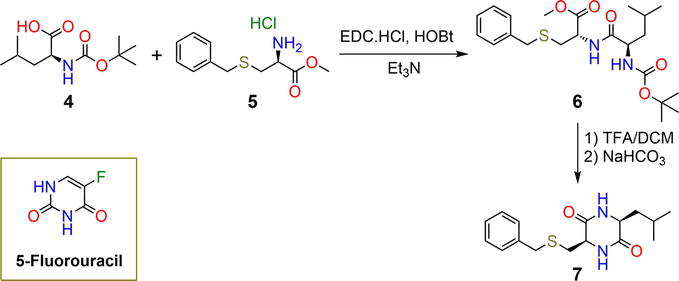
Figure 2.
Synthetic pathway of hydrogelators
Luis
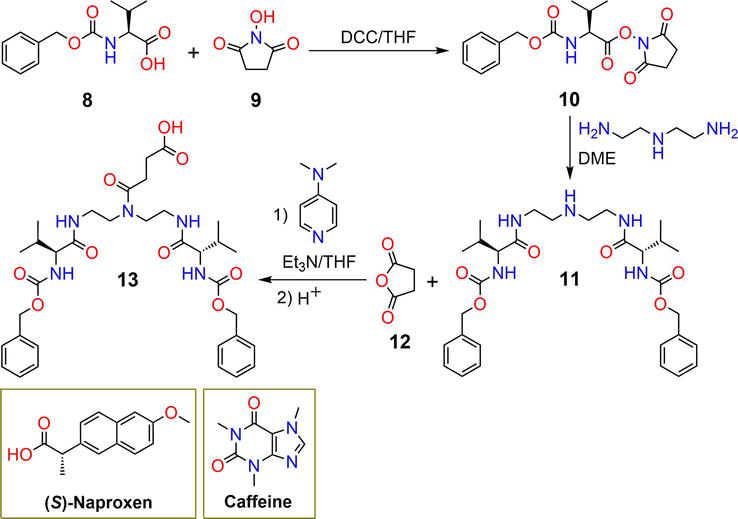
Figure 3.
Synthetic pathway of hydrogel
Compound
Ionic hydrogels are a readily available and cost-effective class of materials that have been widely explored as significant drug delivery systems. Cetylpyridinium salicylate ionogel
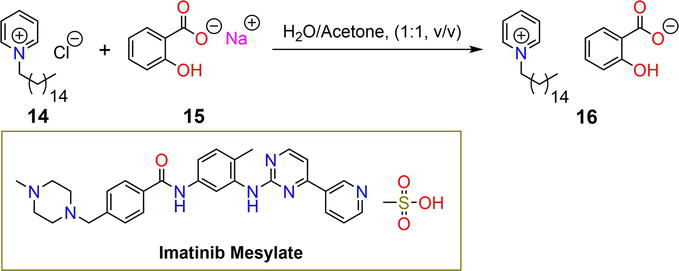
Figure 4.
Synthetic pathway of ionogel
DuttKonar
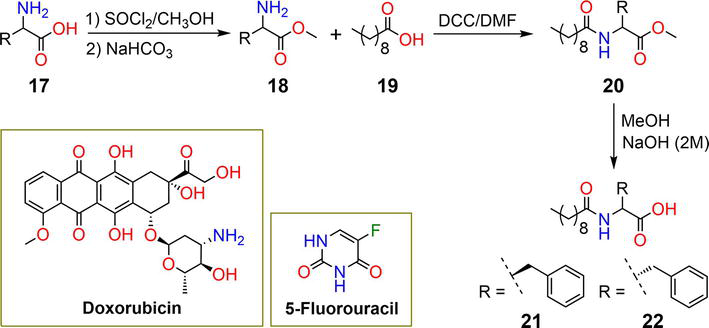
Figure 5.
Synthetic pathway of hydrogels
Compounds
Functionalized calixarene
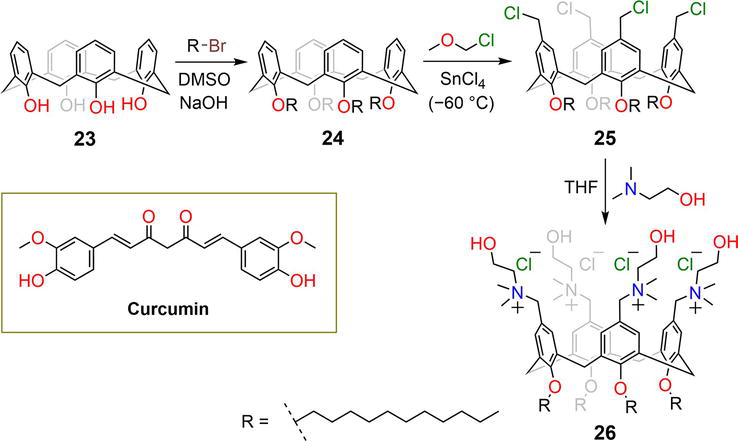
Figure 6.
Synthetic pathway of functionalized calixarene derivative
The tetrachloromethyl-
Gamble
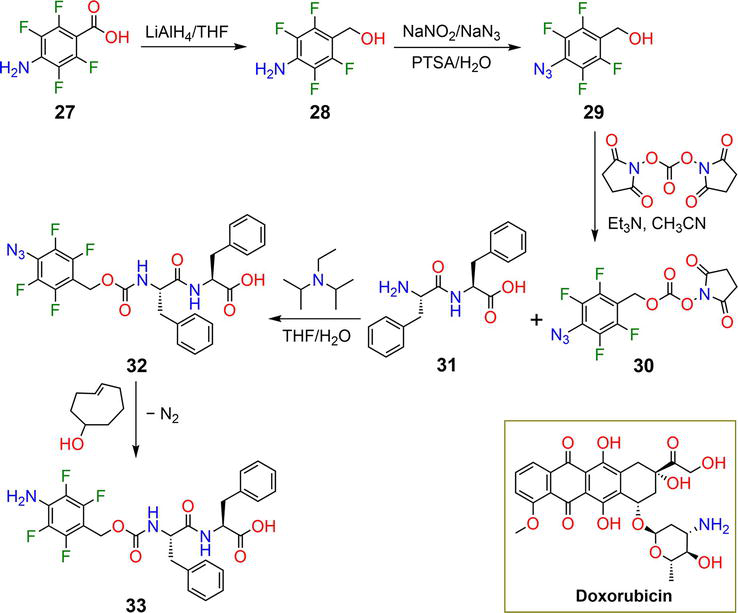
Figure 7.
Synthetic pathway of hydrogelator
Hydrogelator
The reaction of the nucleoside guanosine
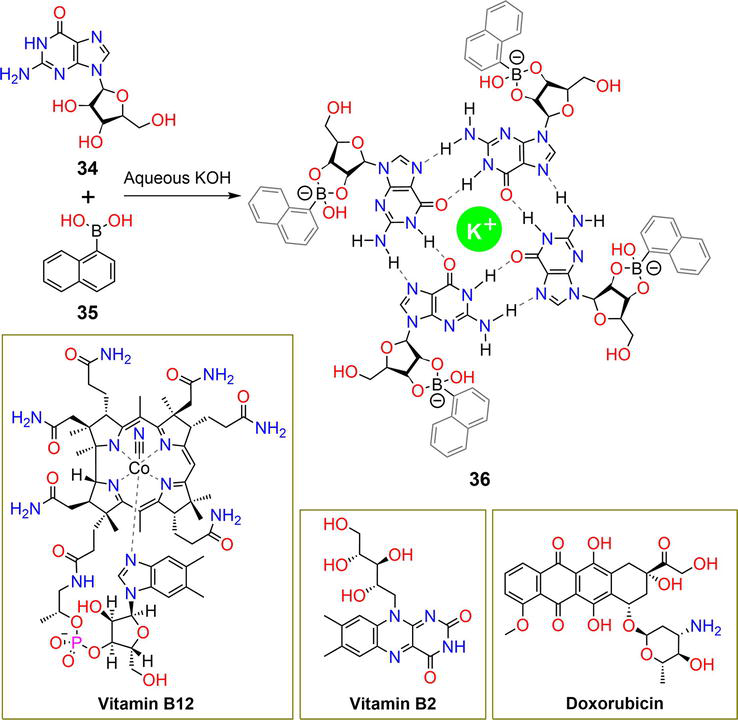
Figure 8.
Synthetic pathway of hydrogelator
The hydrogel matrices released 30% and 60% of vitamin B12 and vitamin B2 after 40 hours, respectively, and the complete release of all entrapped vitamins was observed after 94 hours. At an acidic pH of 4.8, vitamin B12 and vitamin B2 were released in amounts of 82% and 75%, respectively. At pH 7.4 and 4.8, the DOX drug achieved 40% and 76% release, respectively. The pH-responsive behavior of the hydrogel was attributed to the breakage of hydrogen bonding interactions and boronate ester bonds.
Nayak
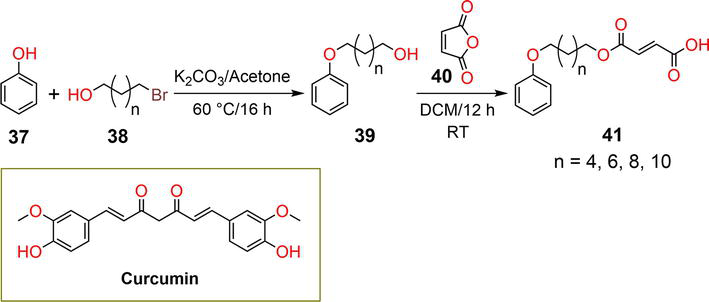
Figure 9.
Synthetic pathway of hydrogelator
Huang
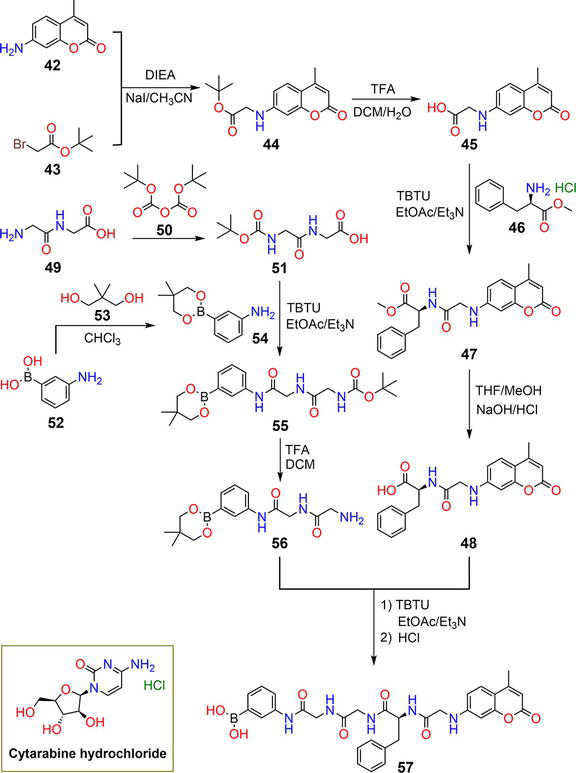
Figure 10.
Synthetic pathway of hydrogelator
DuttKonar
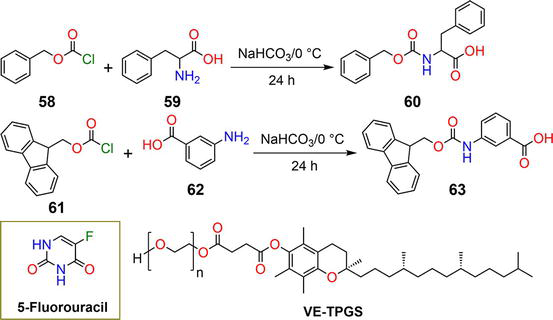
Figure 11.
Synthetic pathway of hydrogelators
Gelators
Bajaj
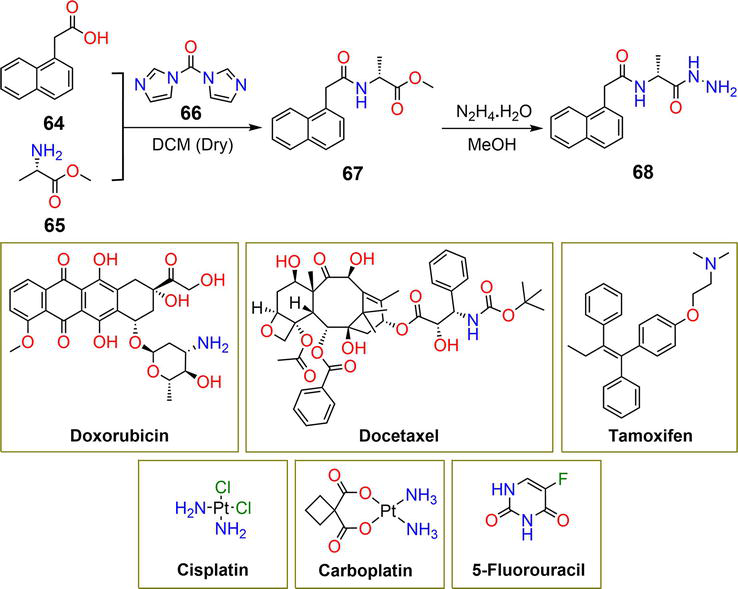
Figure 12.
Synthetic pathway of hydrogelator
Cisplatin and carboplatin showed minimum loading efficiency despite their relatively low molecular weights due to the absence of π-π stacking interactions and disruption of hydrogen bonding of the gelator by the amino groups [44]. The hydrazide group existing in the structural framework of gelator
Compound
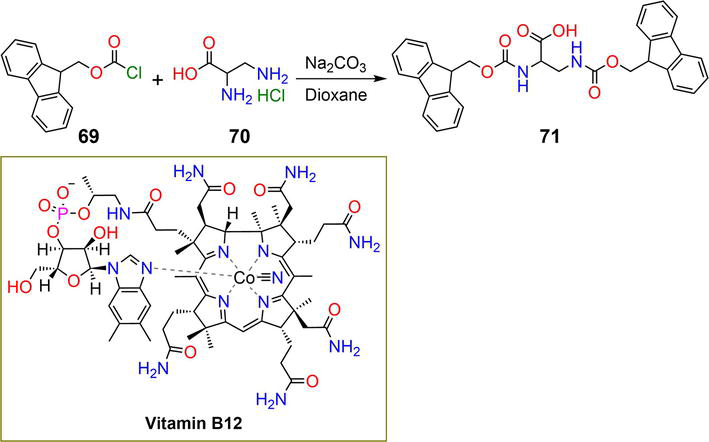
Figure 13.
Synthetic pathway of hydrogelator
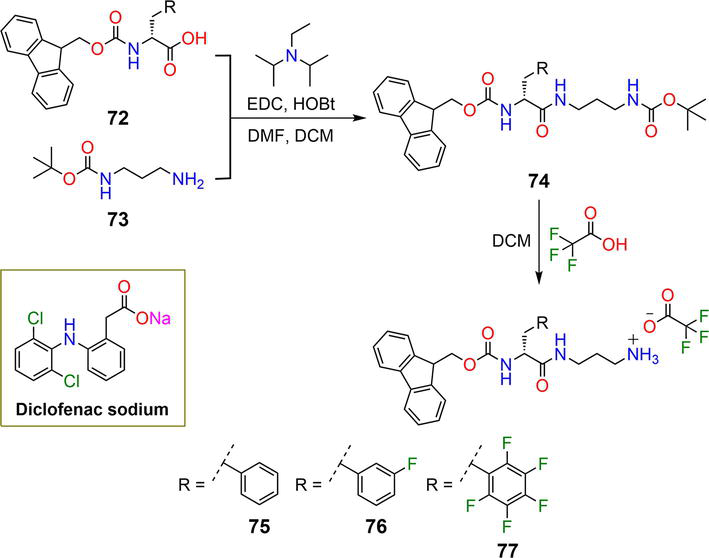
Figure 14.
Synthetic pathway of hydrogelators
Gelators
The drug-loaded mixtures were incubated with a phosphate-buffered saline solution at a pH of 7 and a temperature of 37°C. All the hydrogels displayed slow and sustained drug release over the course of time in the order
Drug loading can also be achieved by linking drug molecules to the gelators, while release can be triggered using appropriate stimuli. Kundu
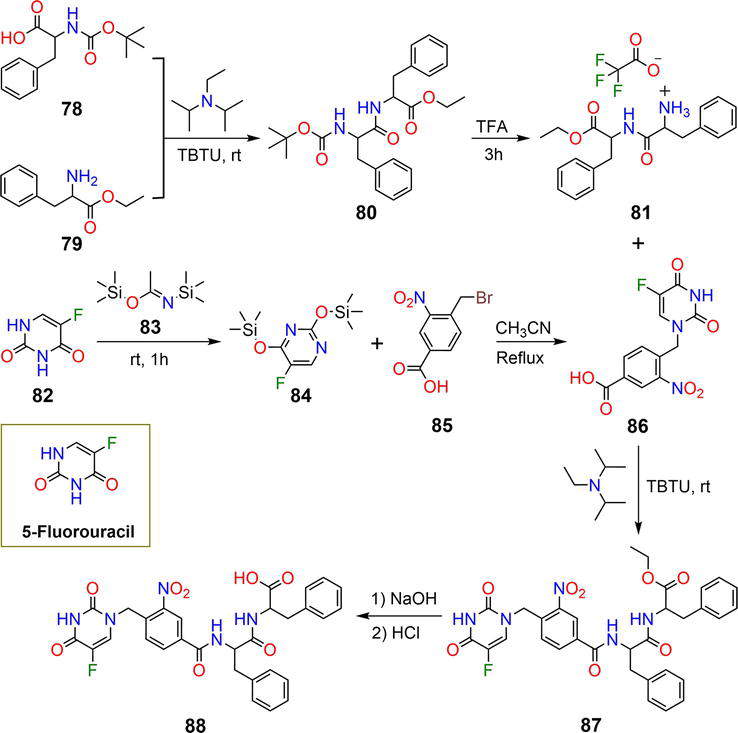
Figure 15.
Synthetic pathway of hydrogelator
The reaction of the anticancer drug 5-fluorouracil
Pianowski
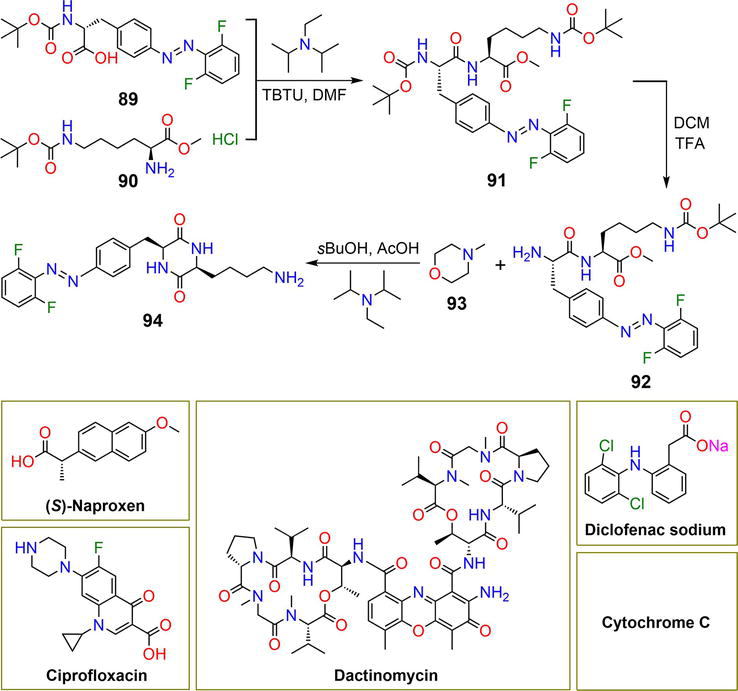
Figure 16.
Synthetic pathway of hydrogelator
Compound
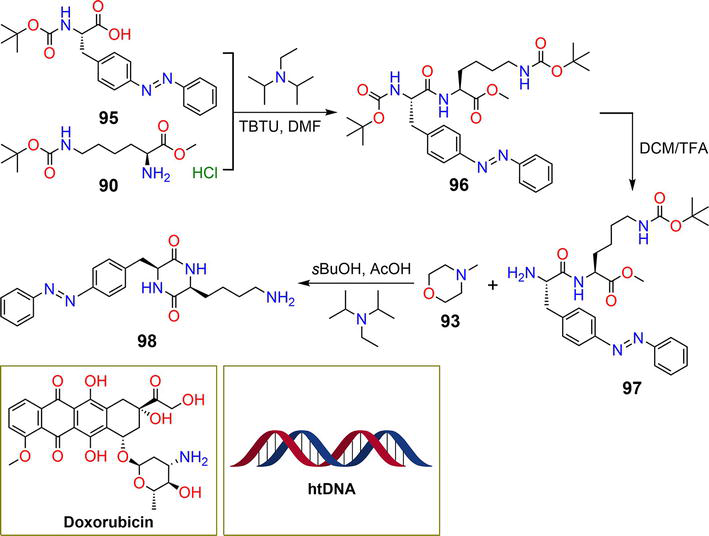
Figure 17.
Synthetic pathway of hydrogelator
Hydrolysis of compound
In summary, Table 1 presents an overview of the hydrogelators discussed in this chapter, along with their CGCs, the substances that can be trapped within the hydrogel matrix, and the conditions under which drug release occurs.
Entry | CGC | Cargos | Stimulus | References |
---|---|---|---|---|
3 | — | DOX | pH | [28, 29] |
7 | 0.05% | 5-FU | pH | [30] |
13 | 1 mg mL−1 | ( | pH | [32] |
16 | 4.7% | IM | Franz diffusion | [34] |
21, 22 | 0.01% | 5-FU, DOX | pH | [35] |
26 | — | CUR | pH | [37] |
32 | 0.02–0.1 wt% | DOX | Bioorthogonal trigger | [38] |
36 | — | B12, B2, DOX | pH | [39] |
41 | 1.3 | CUR | Enzyme/pH | [40] |
57 | 2.7 mg mL−1 | ARA-C | Light | [41] |
60 | 0.02% | 5-FU | pH | [42] |
63 | 0.05% | 5-FU | pH | [42] |
68 | 1.0% | DOX, DTX, TAM, CP, CB, 5-FU | pH | [43] |
71 | 0.3 wt% | B12 | pH | [46] |
75, 76, 77 | 2.5, 2.5, 5 mM | DCF | pH | [47, 48] |
88 | 2 wt% | 5-FU | Light | [49] |
94 | 3.0 wt% | ( | Light | [27] |
98 | 1.0% | DOX, htDNA | Light | [50] |
Table 1.
Abbreviations: DOX, Doxorubicin; 5-FU, 5-Fluorouracil; (
5. Conclusions and prospects
LMWHs have emerged as promising materials with potential applications in various research areas. The unique properties of LMWHs, such as cost-effective preparation, easy functionalization, biocompatibility, low toxicity, and ability to respond to external stimuli, make them ideal candidates for different biomedical applications. LMWHs have shown great potential as drug delivery vehicles for a variety of drugs. However, there are several obstacles that should be addressed in order to fully exploit their promise in drug delivery applications. One of the major drawbacks of LMWHs is their weak mechanical strength, which can be tuned by modifying the structure of hydrogelators, such as incorporating more functional motifs, to promote and strengthen noncovalent interactions. Co-assembly can also reinforce the mechanical properties of LMWHs. The stability of LMWHs under physiological conditions or in response to external stimuli is a crucial factor that should be considered during the development of hydrogels intended for drug delivery applications. LMWHs possess limited drug-loading capacity and, in some circumstances, lack targeted drug delivery. Therefore, the development of more sophisticated strategies for improving the drug-loading potential of LMWHs is an urgent necessity. Moreover, drug release from LMWHs may also be inefficient, leading to suboptimal therapeutic effects. Thus, careful design of the structure of the hydrogelators and selection of the triggers are crucial for achieving optimal drug release. Finally, sustained and slow releases of drugs are two vital aspects to be accomplished for efficient drug delivery using LMWHs.
We hope that this chapter will spark further investigations into the synthetic approaches of LMWHs and lead to the discovery of novel gelators with potential applications in drug delivery.
References
- 1.
Patil SP, Jeong HS, Kim BH. A low-molecular-weight supramolecular hydrogel of riboflavin bolaamphiphile for VEGF-siRNA delivery. Chemical Communications. 2012; 48 (71):8901-8903 - 2.
Patil SP, Kim S-H, Jadhav JR, Lee J-H, Jeon EM, Kim K-T, et al. Cancer-specific gene silencing through therapeutic siRNA delivery with B vitamin-based nanoassembled low-molecular-weight hydrogelators. Bioconjugate Chemistry. 2014; 25 :1517-1525 - 3.
Sedghiniya S, Soleimannejad J, Blake AJ. A low molecular weight Zr(IV) metallogel for protein delivery. Materials Today Communication. 2021; 27 :102448 - 4.
Jagrosse ML, Agredo P, Abraham BL, Toriki ES, Nilsson BL. Supramolecular phenylalanine-derived hydrogels for the sustained release of functional proteins. ACS Biomaterials Science & Engineering. 2023; 9 (2):784-796 - 5.
McCloskey AP, Lee M, Megaw J, McEvoy J, Coulter SM, Pentlavalli S, et al. Investigating the in vivo antimicrobial activity of a self-assembling peptide hydrogel using a galleria mellonella infection model. ACS Omega. 2019;4 :2584-2589 - 6.
Reddy SM, Shanmugam G, Duraipandy N, Kiran MS, Mandal AB. An additional fluorenylmethoxycarbonyl (Fmoc) moiety in di-Fmoc-functionalized L-lysine induces pH-controlled ambidextrous gelation with significant advantages. Soft Matter. 2015; 11 (41):8126-8140 - 7.
Bernet A, Behr M, Schmidt H-W. Supramolecular hydrogels based on antimycobacterial amphiphiles. Soft Matter. 2012; 8 :4873-4876 - 8.
Cross ER, Coulter SM, Fuentes-Caparros AM, McAulay K, Schweins R, Laverty G, et al. Tuning the antimicrobial activity of low molecular weight hydrogels using dopamine autoxidation. Chemical Communications. 2020; 56 (58):8135-8138 - 9.
Zhao X, Zhang H, Gao Y, Lin Y, Hu J. A simple injectable moldable hydrogel assembled from natural glycyrrhizic acid with inherent antibacterial activity. ACS Applied Bio Materials. 2020; 3 (1):648-653 - 10.
Aldilla VR, Chen R, Martin AD, Marjo CE, Rich AM, Black DS, et al. Anthranilamide-based short peptides self-assembled hydrogels as antibacterial agents. Scientific Reports. 2020; 10 (1):770 - 11.
Zhao Q , Zhao Y, Lu Z, Tang Y. Amino acid-modified conjugated oligomer self-assembly hydrogel for efficient capture and specific killing of antibiotic-resistant bacteria. ACS Applied Materials & Interfaces. 2019; 11 (18):16320-16327 - 12.
Rizzo C, Arrigo R, Dintcheva NT, Gallo G, Giannici F, Noto R, et al. Supramolecular hydro- and ionogels: A study of their properties and antibacterial activity. Chemistry - A European Journal. 2017; 23 (64):16297-16311 - 13.
Tang Q , Plank TN, Zhu T, Yu H, Ge Z, Li Q , et al. Self-assembly of metallo-nucleoside hydrogels for injectable materials that promote wound closure. ACS Applied Materials & Interfaces. 2019; 11 (22):19743-19750 - 14.
Wei Q , Chang Y, Ma G, Zhang W, Wang Q , Hu Z. One-pot preparation of double network hydrogels via enzyme-mediated polymerization and post-self-assembly for wound healing. Journal of Materials Chemistry B. 2019; 7 (40):6195-6201 - 15.
Liang C, Zhang L, Zhao W, Xu L, Chen Y, Long J, et al. Supramolecular nanofibers of drug-peptide amphiphile and affibody suppress HER2+ tumor growth. Advanced Healthcare Materials. 2018; 7 (22):e1800899 - 16.
Citossi F, Smith T, Lee JB, Segal J, Gershkovich P, Stocks MJ, et al. Self-assembling benzothiazole-based gelators: A mechanistic understanding of in vitro bioactivation and gelation. Molecular Pharmaceutics. 2018;15 (4):1578-1586 - 17.
Bhagat SD, Srivastav A. Amphiphilic phenylalanine derivatives that temporally generate reactive oxygen species from water in the presence of Au(III) ions. Biomaterials Science. 2020; 8 (17):4750-4756 - 18.
Zanna N, Focaroli S, Merlettini A, Gentilucci L, Teti G, Falconi M, et al. Thixotropic peptide-based physical hydrogels applied to three-dimensional cell culture. ACS Omega. 2017; 2 (5):2374-2381 - 19.
Tang JD, Mura C, Lampe KJ. Stimuli-responsive, pentapeptide, nanofiber hydrogel for tissue engineering. Journal of the American Chemical Society. 2019; 141 (12):4886-4899 - 20.
Suga T, Osada S, Narita T, Oishi Y, Kodama H. Promotion of cell adhesion by low-molecular-weight hydrogel by Lys based amphiphile. Materials Science and Engineering: C. 2015; 47 :345-350 - 21.
Talloj SKM, Mohammed M, Lin H-C. Construction of self-assembled nanostructure-based tetraphenylethylene dipeptides: Supramolecular nanobelts as biomimetic hydrogels for cell adhesion and proliferation. Journal of Materials Chemistry B. 2020; 8 (33):7483-7493 - 22.
Dessane B, Smirani R, Bougueon G, Kauss T, Ribot E, Devillard R, et al. Nucleotide lipid-based hydrogel as a new biomaterial ink for biofabrication. Scientific Reports. 2020; 10 (1):2850 - 23.
Chu NT, Chakravarthy RD, Shih NC, Lin YH, Liu YC, Lin JH, et al. Fluorescent supramolecular hydrogels self-assembled from tetraphenylethene (TPE)/single amino acid conjugates. RSC Advances. 2018; 8 (37):20922-20927 - 24.
Nour HF, El Malah T, Khattab TA, Olson MA. Template-assisted hydrogelation of a dynamic covalent polyviologen-based supramolecular architecture via donor-acceptor interactions. Materials Today Chemistry. 2020; 17 :100289 - 25.
Colquhoun C, Draper ER, Eden EG, Cattoz BN, Morris KL, Chen L, et al. The effect of self-sorting and co-assembly on the mechanical properties of low molecular weight hydrogels. Nanoscale. 2014; 6 (22):13719-13725 - 26.
Basak S, Singh I, Banerjee A, Kraatz H-B. Amino acid-based amphiphilic hydrogels: Metal ion induced tuning of mechanical and thermal stability. RSC Advances. 2017; 7 :14461-14465 - 27.
Karcher J, Pianowski ZL. Photocontrol of drug release from supramolecular hydrogels with green light. Chemistry - A European Journal. 2018; 24 (45):11605-11610 - 28.
Noteborn WEM, Vittala SK, Torredemer MB, Maity C, Versluis F, Eelkema R, et al. Switching the mode of drug release from a reaction-coupled low-molecular-weight gelator system by altering its reaction pathway. Biomacromolecules. 2023; 24 (1):377-386 - 29.
Poolman JM, Boekhoven J, Besselink A, Olive AG, van Esch JH, Eelkema R. Variable gelation time and stiffness of low-molecular-weight hydrogels through catalytic control over self-assembly. Nature Protocols. 2014; 9 (4):977-988 - 30.
Ghosh S, Nag S, Saha KD, Banerji B. S -benzyl cysteine based cyclic dipeptide super hydrogelator: Enhancing efficacy of an anticancer drug via sustainable release. Journal of Peptide Science. 2022;28 (8):e3403 - 31.
Wadhavane PD, Gorla L, Ferrer A, Altava B, Burguete MI, Izquierdo MÁ, et al. Coordination behaviour of new open chain and macrocyclic peptidomimetic compounds with copper(ii). RSC Advances. 2015; 5 :72579-72589 - 32.
Valls A, Isabel Burguete MK, Kuret L, Altava B, Luis SV. Open chain pseudopeptides as hydrogelators with reversible and dynamic responsiveness to pH, temperature and sonication as vehicles for controlled drug delivery. Journal of Molecular Liquids. 2022; 348 :118051 - 33.
Bica K, Rijksen C, Nieuwenhuyzen M, Rogers RD. In search of pure liquid salt forms of aspirin: Ionic liquid approaches with acetylsalicylic acid and salicylic acid. Physical Chemistry Chemical Physics. 2010; 12 (8):2011-2017 - 34.
Kuddushi M, Patel NK, Rajput S, El Seoud OA, Mata JP, Malek NI. Temperature-responsive low molecular weight ionic liquid based gelator: An approach to fabricate an anti-cancer drug-loaded hybrid ionogel. ChemSystemsChem. 2020; 2 (5):e1900053 - 35.
Mehra RR, Basu A, Christman RM, Harjit J, Mishra AK, Tiwari AK, et al. Mechanoresponsive, proteolytically stable and biocompatible supergelators from ultra short enantiomeric peptides with sustained drug release propensity. New Journal of Chemistry. 2020; 44 :6346-6354 - 36.
Rodik RV, Anthony A-S, Kalchenko VI, Mélya Y, Klymchenko AS. Cationic amphiphilic calixarenes to compact DNA into small nanoparticles for gene delivery. New Journal of Chemistry. 2015; 39 :1654-1664 - 37.
Granata G, Petralia S, Forte G, Conoci S, Consoli GML. Injectable supramolecular nanohydrogel from a micellar self-assembling calix[4]arene derivative and curcumin for a sustained drug release. Materials Science and Engineering: C. 2020; 111 :110842 - 38.
Dadhwal S, Fairhall JM, Hook S, Gamble AB. Tetrafluoroaryl azide as an N-terminal capping group for click-to-dissolve diphenylalanine hydrogels. RSC Advances. 2020; 10 (16):9234-9244 - 39.
Ghosh T, Biswas A, Gavel PK, Das AK. Engineered dynamic boronate ester-mediated self-healable biocompatible G-quadruplex hydrogels for sustained release of vitamins. Langmuir. 2020; 36 (6):1574-1584 - 40.
Kumar BA, Nayak RR. Supramolecular phenoxy-alkyl maleate-based hydrogels and their enzyme/pH-responsive curcumin release. New Journal of Chemistry. 2019; 43 :5559-5567 - 41.
Liu Q , Wang H, Li G, Liu M, Ding J, Huang X, et al. A photocleavable low molecular weight hydrogel for light-triggered drug delivery. Chinese Chemical Letters. 2019; 30 :485-488 - 42.
Tiwari P, Rajagopalan R, Moin M, Soni R, Trivedi P, DuttKonar A. Can self-assembled hydrogels composed of aromatic amino acid derivatives function as drug delivery carriers? New Journal of Chemistry. 2017; 41 :308-315 - 43.
Singh M, Kundu S, Reddy M, Sreekanth V, Motiani RK, Sengupta S, et al. Injectable small molecule hydrogel as a potential nanocarrier for localized and sustained in vivo delivery of doxorubicin. Nanoscale. 2014;6 :12849-12855 - 44.
Gupta S, Singh M, Reddy M. A, Yavvari PS, Srivastava a, Bajaj a, interactions governing the entrapment of anticancer drugs by low-molecular-weight hydrogelator for drug delivery applications. RSC Advances. 2016; 6 :19751-19757 - 45.
Nishikawa T, Urabe D, Isobe M. Syntheses of N -acylisoxazolidine derivatives, related to a partial structure found in zetekitoxin AB, a golden frog poison. Heterocycles. 2009;79 :379-385 - 46.
Arokianathan JF, Ramya KA, Janeena A, Deshpande AP, Ayyadurai N, Leemarose A, et al. Non-proteinogenic amino acid based supramolecular hydrogel material for enhanced cell proliferation. Colloids and Surfaces B: Biointerfaces. 2020; 185 :110581 - 47.
Rajbhandary A, Raymond DM, Nilsson BL. Self-assembly, hydrogelation, and nanotube formation by cation-modified phenylalanine derivatives. Langmuir. 2017; 33 (23):5803-5813 - 48.
Raymond DM, Abraham BL, Fujita T, Watrous MJ, Toriki ES, Takano T, et al. Low molecular weight supramolecular hydrogels for sustained and localized in vivo drug delivery. ACS Applied Bio Materials. 2019; 2 (5):2116-2124 - 49.
Das S, Horo H, Goswami U, Kundu LM. Synthesis of a peptide conjugated 5-fluorouracil gelator prodrug for photo-controlled release of the antitumor agent. ChemistrySelect. 2019; 4 (22):6778-6783 - 50.
Pianowski ZL, Karcher J, Schneider K. Photoresponsive self-healing supramolecular hydrogels for light-induced release of DNA and doxorubicin. Chemical Communications. 2016; 52 (15):3143-3146