Iron ore/oxide reduction pattern for common reducing agents in gaseous forms.
Abstract
The blast furnace and direct reduction processes have been the major iron production routes for various iron ores (i.e. goethite, hematite, magnetite, maghemite, siderite, etc.) in the past few decades, but the challenges of maintaining the iron and steel-making processes are enormous. The challenges, such as cumbersome production routes, scarcity of metallurgical coke, high energy demands, and high cost of production, cannot be overemphasized. This study provides a systematic overview of the different ironmaking routes, their operational limitations and proper sound future perspectives to mitigate the challenges involve based on iron production demands in the modern-day metallurgical process. Subsequently, strategic ways toward improving the production efficiency and product quality of metallic iron produced in the recent iron processing routes were suggested. The study reiterated that the non-contact direct reduction and reduction-smelting routes are the faster ironmaking and steelmaking processes that can utilize alternative energy sources efficiently with little or no carbon deposition. Both processes also have promising features based on their requirements in terms of fewer energy demands, time-saving, cost-effectiveness, and operational efficiency. Thus, in today’s iron and steelmaking processes, non-contact direct reduction and reduction-smelting processes remain viable alternative iron production routes.
Keywords
- iron ore
- blast furnace
- direct reduction
- smelting process
- ironmaking and steelmaking
1. Introduction
1.1 Study background
The challenges associated with iron ore processing have resulted in rigorous research of new processing techniques in the iron and steelmaking process, considering their diverse applications in the construction and manufacturing industries. Iron ore consists of various elements within its metal matrix with the presence of silicate and aluminate as the gangue materials. A trace amount of manganese, titanium, nickel, etc. can be found within their microstructure. Iron ore processing has its root in hematite (α-Fe2O3), maghemite (γ-Fe2O3), magnetite (Fe3O4), goethite (α-FeOOH), siderite (Fe2CO3), etc. which can be prepared as nuggets for major feeds in the blast furnace as well as other conventional iron-making processes [1]. The focus of most research from the works of literature revolves around the production of iron metals through recycling or improving upon the existing method of production in the iron and steelmaking industry [2, 3, 4]. These methods include the Blast furnace/Blast Oxygen Furnace [5, 6], Direct Reduction Method [7], Indirect Reduction Method [8, 9], and Reducer-Smelter Process [10, 11, 12]. Thus, it is, therefore crucial to know that this processing technique has proven to have produced quality iron but not without a high cost of production, increased environmental pollution, and cumbersome production process [13, 14]. Therefore, an in-depth approach to producing metallic iron that is cost-effective, low risk of environmental pollution, and easy production route becomes imperative [15, 16, 17]. Thus, this paper attempts to investigate the challenges militating against the various iron extraction routes in the modern-day iron and steel-making process, Special attention was paid to the analysis of the metallurgical operation behind the conventional iron and steelmaking process, while an extensive overview of the merits, advantages, limitations and future perspective on improvement strategies for the conventional iron and steel production routes were also substantiated.
Also, a new iron extraction technique known as the non-contact direct reduction and smelting process was introduced as a decarburization mechanism and preventive approach to mitigate the direct interaction of harmful elements such as sulfur and phosphorus in conventional iron and steelmaking processes. The processing parameter for the production of direct reduced iron (DRI) in the conventional ironmaking process was also meticulously investigated to mitigate the effect of carbide formation across the intra-granular surface of the DRI which is likely responsible for environmental pollution due to high carbon deposit from the iron and steel production in modern-day metallurgical processes. The carbon-bearing substance and non-carbonaceous materials utilized for this study are non-coking coal, charcoal, biomass, methane (CH4), hydrogen (H2), and carbon monoxide (CO).
2. The iron ore/iron oxide reduction technique
Iron ore or iron oxide reduction is the process of removing oxides from ferrous materials. Some of these ferrous materials include hematite, magnetite, siderite, goethite, etc. Several processes have been postulated in the works of literature. Among the reduction methods of iron oxides in ironmaking processes are direct reduction, blast furnace/blast oxygen furnace, and smelting-reduction process. Reduction of iron oxide involves the upgrade of iron ore in pellets, lumps, or sintered form under a highly thermal-charged atmosphere in the presence of carbonaceous materials [18, 19], agric waste [20], or reducer gases [21].
2.1 Direct reduction process
Direct Reduced Iron (DRI) is the product of the reduction of iron ores (lumps, pellets, or fines) in solid-state by gaseous or carbonaceous substances [22, 23]. The reduction reaction temperature range generally occurs between 450 to 1300°C. The DR technique is a broad group of processes based on different feedstocks, furnaces, and reducing agents. Its common operation principle entails the removal of oxygen (reduction) from iron ores in the solid state, unlike the blast furnace method that comprises both solid and liquid state iron oxide reduction. Natural gas (in some cases coal) is used as a reducing agent in this method of iron extraction. In the year 2000, about 92.6% of DRI was based on natural gas processed in shaft furnaces, retorts, and fluidized bed reactors [24]. The metallization rate of the end product ranges from 85–95% (often even higher). The product from this process is called DRI or sponge iron or hot briquette iron (HBI), which is a highly metalized solid that still contains gangue minerals, and hence needs further purification [25].
The direct reduction method was adopted by Delta Steel Plant in Nigeria, while they were still in operation [26]. Most steel plants across the globe also adopt this method, by making use of ferrous scraps. Figure 1 provides a layout of the direct reduction of the process, while Figure 2 provides the general schematic flow for performing the direct reduction process of iron ore/oxide in a carbonaceous atmosphere from lump charcoal/non-coking coal with the view to further give the ironmaking process a more robust perspective during the production of pure iron.
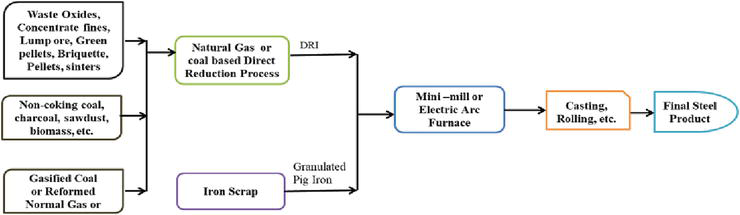
Figure 1.
Schematics of iron and steel production routes by direct reduction method [
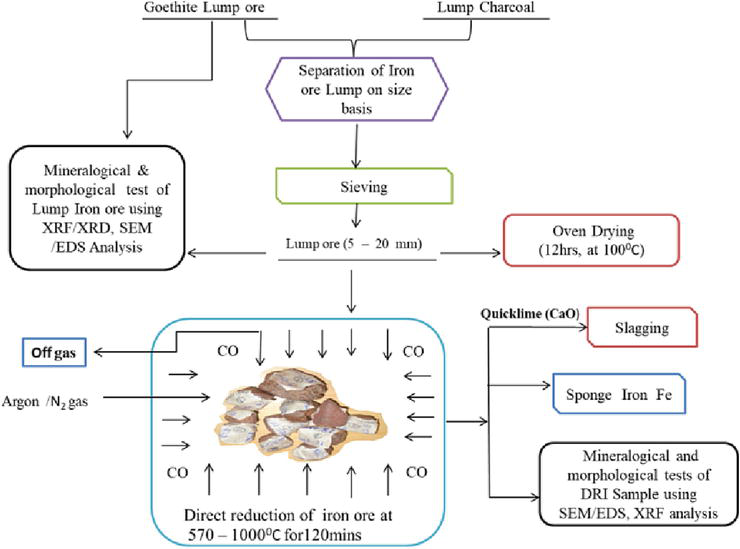
Figure 2.
Schematics of an experiment flow chart of direct reduction of iron ore/oxide.
In this process, iron is extracted from its ore at a temperature below the melting points of the materials involved. The method is used mostly in special circumstances, often linked to cheap supplies of natural gas. Gojic et al. [28] have identified different challenges associated with the direct reduction process of which MIDREX (named after its developer, a division of the Midland-Ross Corporation) is the world’s leading technology.
Thus, the common challenge which is peculiar to the direct reduction process of iron ore/oxide is the need for a regular supply of natural gas (in the case of gaseous reductants), which may be distorted by the incessant attack on gas pipelines in some parts of the world. Gangue remains in the sponge-like product, known as DRI), which must be removed in subsequent ironmaking and steelmaking process, hence more cost is accrued. Only high-grade ores and pellets made from super-concentrated iron ore (above 66% iron) are suitable for the DR iron-making process, therefore beneficiation of iron ore samples is needed and this may also increase the cost of production.
2.2 Indirect reduction process
This involves the production of iron using other sources of reductant substances such as carbon II oxide, methane, hydrogen gas, etc. it comprises of solid-gaseous reduction process of iron ore other than coke. It is majorly the strategy for producing metallic iron as an alternative route to the blast furnace or blast oxygen furnace and electric arc furnaces. This also involves the use of pure carbon monoxide (CO), hydrogen (H2), methane (CH4) gas, etc. as the major reductants. For the major gaseous reductants (i.e. CO, H2, and CH4) in a DR process, the following three reduction reactions can be written as shown in Table 1.
Iron Ore/IronOxide | Reduction with CO | Reduction with H2 | Reduction with CH4 |
---|---|---|---|
Hematite | 3Fe2O3+ CO → 2Fe3O4+ CO2 | 3Fe2O3+ H2 → 2Fe3O4+ H2O | 12Fe2O3+ CH4 → 2Fe3O4+ CO2 + 2H2O |
Magnetite | Fe3O4+ CO → 3FeO + CO2 | Fe3O4+ H2 → 3FeO + H2O | 3Fe3O4+ CH4 → 3Fe + CO2 + 2H2O |
Wustite | FeO + CO → Fe + CO2 | FeO + H2 → Fe + H2O | Fe + CH4 → Fe + CO2 + H2O |
Table 1.
The indirect reduction approach can also be expensive as it is unadvisable for usage in iron and steelmaking processes at an industrial scale. Majorly, the indirect reduction method can only be sustainable when carried out on a laboratory scale. Though, iron nugget produced from this approach tends to be gangue free and often free of radical elements such as sulfur and phosphorus which may interfere with the chemical activities within the microstructure phase of the heat-treated iron ore. Furthermore, the activity of noble gases such as argon is employed in the indirect iron reduction process to mitigate the interference of other external gases from taking part in the reduction process. The reduction reaction between solid iron ore and carbon monoxide entails the removal of oxygen from higher oxide of iron under strict experimental conditions. The reaction can be a solid–gas or liquid–gas reaction where the release of carbondioxide gas and lesser oxides of iron are acquired as the product of the reduction process.
2.3 Non-contact direct reduction (NDR) process
The Non-contact Direct Reduction (NDR) process is a novel approach aimed to mitigate the effect of excess carbon deposit on DRI which is most common in the iron product produced via other conventional iron-making processes. This approach tends to also bypass the idea of pelletization, communition, and hot briquette preparation of iron ore-reductants composites which have shown to have high economic implications, are energy-demanding, time-consuming, etc. Ogbezode et al. [29] introduce the concept of the ironmaking technique the NDR method by investigating the reduction behavior of goethite ore using carbon monoxide gas from the wood charcoal atmosphere. The result revealed a DRI with a metallization degree of over 93% was achieved at an overall reduction temperature of 1000°C. Ogbezode et al. [30] also performed an NDR process on selected goethite-hematite ore under isothermal conditions using CO/CO2 atmosphere from wood charcoal. The kinetics of the reaction and rate-controlling resistances of the process was monitored at different roasting temperatures and reaction rate. The study affirmed that the presence of an ash layer deposit from the charcoal which is transported by gas penetration from the burned charcoal forms an interstitial layer on the DRI and was responsible to have slowed down the rate of reaction between iron ore and the reducing gas. The advantage of this direct reduction method is that the DRI produced via the process does not accommodate any carbon deposits and sulfur within the metallic iron phase microstructure.
The limitation of the non-contact reduction method of iron ore is the presence of gangue materials that may be present as impurities within the metal matrix of the DRI. Thus, for effective and optimum performance, the DRI produced by the non-contact direct reduction method should be smelted to eliminate gangue minerals from its metal structure using quicklime (CaO) or limestone (CaCO3) at a smelting temperature above 1400°C. A schematic view of the NDR process is depicted in Figure 3.
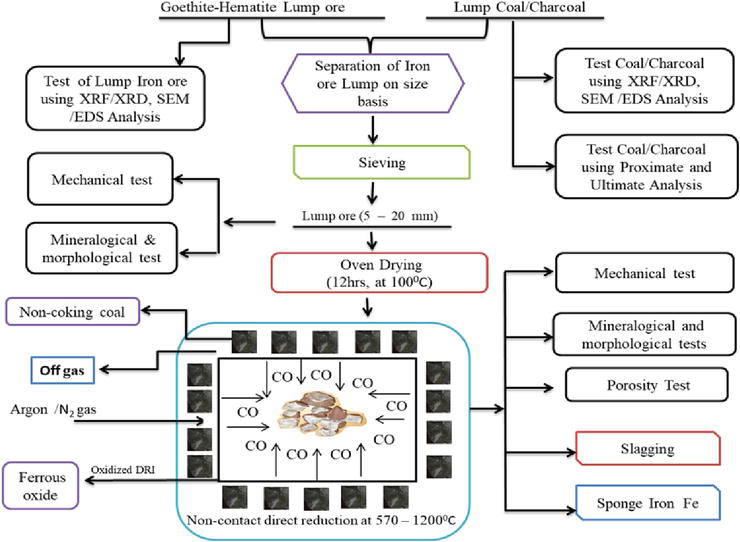
Figure 3.
A schematic view of the non-contact direct reduction process of iron ore/oxide.
2.4 Reduction-smelting process of iron ore/oxide using solid reductants
An alternative ironmaking and steelmaking process that utilizes other sources of energy instead of the conventional metallurgical coke used in blast furnace operations can be referred to as a smelting-reduction process. It is a metallurgical operation with a dual-compartment approach where the pre-reduction and post-reduction process takes place. The pre-reduction chambers utilize iron ore in pellets, lumps, or sintered form to a metallization level of over 90%, in the CO/CO2 atmosphere from any carbon-bearing material at a reduction temperature of between 570–1300°C. Then, the reduced iron ore is then charged into the post-reduction chamber for the smelting process at a temperature above 1400°C. This operation is aimed to actualize a slagging process through the introduction of limestone (CaCO3) or quicklime (CaO). The pure metallic iron condenses, while the gangues are fluxed out as aluminate and silicate compounds known as slags. Liquid iron or pig iron is obtained as the final product which can then be subject to the casting and rolling process to obtain a final product as steel. Figure 4 tries to depict a modification of the Anameric et al. [27] approach for the iron reduction-smelting process, the modification was necessary to mitigate the possible limitations that may affect the process in the long run. For instance, this can be envisaged by introducing a mini-reduction route within the ambiance of the post-reduction route through the use of hot reduction gas by de-oxidation of carbon dioxide gas released from the post-reduction vessel as shown in Figure 5.
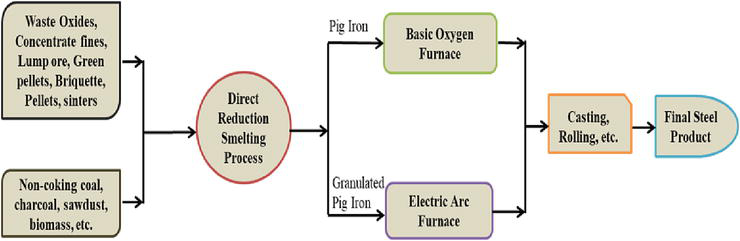
Figure 4.
Schematics of iron and steel production routes by direct reduction-smelting process [
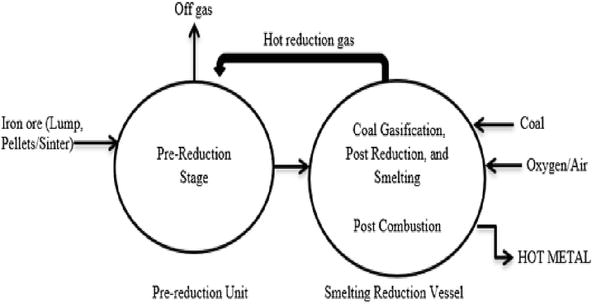
Figure 5.
Layout of a typical smelting reduction process [
In a typical reduction-smelting process, the iron ore will first undergo a solid-state reduction in the pre-reduction unit. The resulting product is then smelted and further reduced in the smelting-reduction vessel where the coal gasification occurs, thus delivering heat and carbon-monoxide-rich hot gas. Coal gasification takes place due to the reaction between oxygen and iron ore in a liquid state by the thermal combustion process. The heat is used to smelt the iron, while the hot gas is transported to the pre-reduction unit to reduce the iron oxides that enter the chambers. Reduced iron oxides (similar to DRI) are in turn transported to the smelting-reduction vessel for the final reduction and smelting process. The carbon-monoxide-rich gas generated in the post-combustion chamber can be further oxidized to generate additional heat for the complete reduction and smelting of the pre-reduced DRI.
The direct reduction process takes advantage of the availability of cheap natural gas and/or hydroelectric power, as well as access to suitable iron ores, and agglomerates [32], whereas the reducer-smelter process takes advantage of the accessibility of non-coking coals or reducer gas with low energy requirements, low operating costs, the ability to respond flexibly to variations in production rate, and low environmental loads, as there will be no coke oven gas leaks. Because the approach does not necessitate the removal of undesirable materials from the iron ore sample, this step can be bypassed during the iron extraction process [33]. Thus, the numerous challenges that accompany the conventional iron ore processing routes cannot be overemphasized. This limitation includes the interference of foreign substances on the metallic, low capital investment, high cost of production, high energy consumption, scarcity of metallurgical coke, etc.
To address these limitations, the smelting-reduction technology must be complemented with a decarburization mechanism inside the furnace, and the use of non-coking coal, charcoal, biomass, etc. as alternative energy sources in the ironmaking routes (see Figure 6). Thus, the advantage of the smelting-reduction ironmaking technology is also due to its operational feasibility, cost competitiveness, and ability to utilize all kinds of iron ore materials in their natural forms (pellets, lump, sinter, etc.). The process can also utilize other reductants (solid, liquid, gas, etc.) as alternative energy sources instead of conventional metallurgical coke [34].
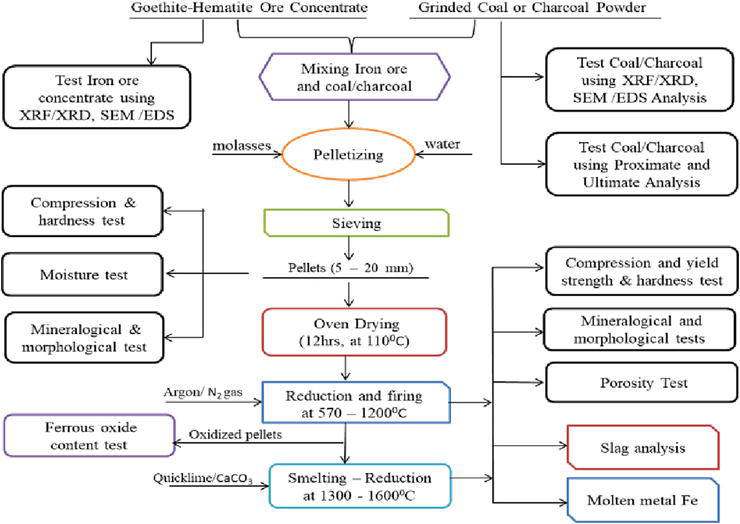
Figure 6.
Schematic flow chart of the reduction-smelting process.
2.5 Reduction-smelting process of iron ore/oxide using gaseous reductants
The quest for the production of iron and steel under clean energy evolution cannot be overemphasized. The negative impact of carbon emissions released from the metallurgical industry on a global scale has given the need to use less harmful reducing gases as a replacement for fossil fuel for the decarburization of the iron and steelmaking process. Heidari et al. [35], explain the need for a green footprint in the metallurgical industry through the use of pure iron oxide and reducer gases. Certain variables such as reduction gas flow rate, H2/CO ratio, diluents concentration (Ar, N2, and He), gas utility, and pressure are among the parameters that foster the production of pure iron using gaseous reductants.
The use of hydrogen unlike CO gas in the metallurgical process has been attempted by many researchers [36, 37], in the quest to produce pure metallic iron in present-day ironmaking technology. The kinetics of the reduction of iron oxide in H2 gas was investigated by Gonoring et al. [38] as a strategy to mitigate the energy consumption rate in conventional metallurgical processes. The study reiterates the need for H2 gas used as an excellent reducing agent in the alternative metallurgical process of iron and steel. Hou et al. [39] conducted a general study on iron oxide reduction kinetics in a hydrogen gas-infested atmosphere under isothermal conditions in a micro-packed bed, influence of diffusion and reduction reaction mechanisms are the major reaction kinetics stages that were enumerated. The magnetite to wustite stage of the reduction process was eliminated leaving behind the hematite to magnetite stage and wustite to the metallic iron stage since the internal and external gas diffusion causes an increase in H2 gas into the inner core of the iron oxide particle provided the reduction process is done at reduction temperature above 570°C. Thus, the intrinsic reduction kinetics of iron oxide can be done in an H2 atmosphere using a micro-packed bed giving reaction kinetics free of internal and external diffusion resistances. Du et al. [40], performed the intermediate reduction on hematite to magnetite ore under low thermal conditions using hydrogen gas. The study acknowledged that the utilization of hydrogen as a reduction rate of hematite to magnetite increases the overall theoretical iron recovery as the rate of hematite conversion increases. Hydrogen remains an efficient reducing agent which has a tremendous effect on iron oxide reduction depending on the reducing flow rate and thermal condition being utilized. Vogl et al. [41] conducted an extensive assessment of the direct reduction of iron oxide void of carbonaceous substances. Ma et al. [42] investigated the reduction behavior of iron oxide pellets in a combined atmosphere of CO, H2, and N2 at a reduction temperature of 900°C. A schematic illustration of the smelting-reduction process of iron oxide using gaseous reductant is depicted in Figure 7.
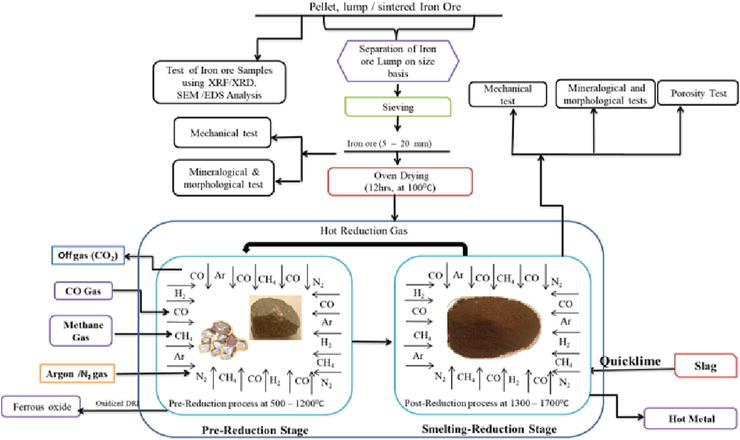
Figure 7.
Schematic flow chart of the reduction-smelting process of iron ore using gaseous reductants.
The study substantiates the thermodynamic response of the iron oxides at different reducer gas flow rates under controlled atmospheric conditions. These works of literature do establish the fact that the assessment outlook of the direct reduction process of iron oxide in conventional steelmaking technology does ride on the premise that the use of gaseous reducing agent iron and steel technology has undoubtedly triggered a metallurgical advancement in iron and steelmaking process with undeniable impact on low economic and environmental implications and optimum overall technological development in the metallurgical industry. Naseri-Seftejanim et al. [43], examined the use of hydrogen in the reduction of hematite ore by the Hydrogen Plasma Smelting Reduction (HPSR) process. The ironmaking technology approach entails the use of hydrogen plasma in the development of a carbon-monoxide-free metallurgical process. In the HPSR process, influencing parameters such as hydrogen utilization degree, iron oxide reduction degree, weight loss, rate of reduction, etc. can be assessed using appropriate experimental conditions. Consequently, the thermodynamic reduction of liquid iron was performed by Naseri-Seftejani et al. [44] using hydrogen plasma. HPSR entails the use of H2 in a plasma state for the reduction of iron ore. The study presents the hematite reduction reaction sequence, hydrogen ionization degree, iron ore particle reaction rate, and reduction temperature as major parameters which affect the overall quality of the molten iron and hydrogen solubility in slag formation produced under severe thermal reduction of the iron ore sample in hydrogen plasma as the most influential of all the reduction parameters. The slag formation from an iron oxide reduced in the HPSR method was investigated by Naseri-Seftejani et al. [45], based on the quest to eliminate the challenges portrayed by CO2 emission in convectional iron and steelmaking technology. The study makes a presentation on reduction degree, operational hydrogen utilization, produced iron, and reduction operational time remains the vital components in slag formation in the HPSR process of iron and steelmaking process under a hydrogenated atmosphere. Thus, the use of the HPSR process in iron and steelmaking technology has proven to be more effective in CO2 elimination. The use of HPSR also produces liquid slag with lower iron oxide content compared to other convectional iron-making processes.
On a general note, the Smelting-Reduction (SR) process of iron oxide can utilize several carbonaceous and non-carbonaceous substances as reducing agents. These reducing agents include metallurgical coke, non-coking coals, charcoal, agric wastes, biomass sawdust, reducer gases (H2, CO/CO2, CH4), etc.
2.6 Iron ore reduction-smelting process using non-coking coals/charcoal as reductants
The use of non-coking coal as an alternative energy source for sponge iron production instead of the conventional metallurgical coke cannot be overemphasized due to the problematic nature that is common to coke making process. The non-coking coal has the advantage of not requiring preprocessing technique as it can be charged into the reactor during sponge iron production. Another interesting fact about non-coking coal remains the fact that it has wider applications in many iron processing reactors or furnaces, compared to that metallurgical coke whose traditional strategy for application is found in the blast furnace. Iron production processes such as FASTMET [46], HISMELT [47], MIDREX [34], COREX [48], Microwave ovens [49], Rotary kiln [50], HIsarna [51], etc. have been used utilized in the works of literature as sponge iron ironmaking processes reactors. Moreover, most of the ironmaking processes have unlaying challenges which are currently been addressed by researchers in the field of materials and metallurgical engineering. The aforementioned iron-making processes which utilize non-coking coal as the alternation energy source are used to produce direct reduced iron DRI which occurs when producing iron metals below their melting point. The DRI also has high impurity content in form of gangue which is mostly locked within the clusters of the DRI crystal structure. Coal based DR process was done on iron ore/coal pellets under axisymmetric thermal conditions [23]. Furthermore, the coal-based reduction under the isothermal conditions on fayalite was conducted by Zhang et al. [52] using thermogravimetric analysis. Li et al. [53] studied the reaction kinetics by carbothermic reduction of fayalite under non-isothermal conditions. Ogbezode et al. [29] performed reduction runs on goethite iron ore under non-isothermal conditions using charcoal. Ogbezode et al. [30] also performed carbothermic reduction and investigated the kinetics behavior of goethite-hematite ore in the CO/CO2 atmosphere. Man et al. [42] also examined changes in iron/ore pellet composite during the carbothermic DR process. Kumar et al. [54], investigated the characteristics of non-coking coal under non-isothermally reduc
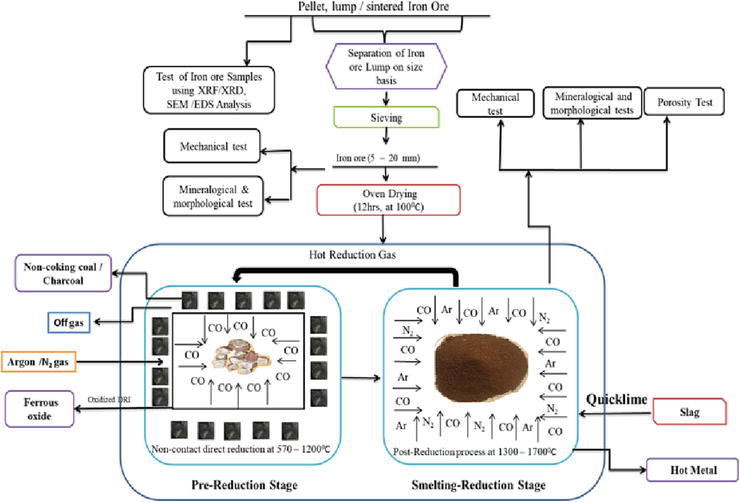
Figure 8.
Schematic flow chart of the reduction-smelting process of iron ore using non-coking or charcoal as reductants.
Therefore, the availability of DRI using non-coking coal as a reducing agent is determined by the availability of iron ore with over 65% Fe quality and the availability of a cheap source of non-coking coal. Due to the operational limitation which accompanies the use of non-coking as the perfect alternative for metallurgical coke used in the blast furnace process, it is necessary to foresee the need to produce pure metallic iron in the direct reduction process. Going forward, the presence of high silicon and alumina content as gangue elements which pose serious limitations on the performance of direct reduced iron begs for the need for the metallurgical industry to source quicklime or limestone in its pure form in slagging operation. Pal et al. [56] performed a development slagging experiment by smelting operation of pellet iron ore using calcined lime instead of limestone and magnesium oxide in a carbonaceous atmosphere. Thus, the use of methane, hydrogen, and pure carbon monoxide gas became necessary in the quest to produce the needed feeds for the production of pure metallic iron.
3. Solid: solid reduction of iron ore by carbon
For solid carbon in a DR process, the following three reduction reactions can be written:
Only a negligible amount of reduction will occur by direct contact of carbon particles with iron oxide particles since such solid-solid reactions are very slow. The actual reduction process occurs through the intermediary of CO.
In the direct reduction process of iron ore where solid carbon is the reductant, self-reducing pellets, briquettes or composites entails iron ore embedded in coal or carbonaceous materials are typically used in blast furnace and direct reduction processes to mitigate the effect of carbon consumption for better productivity and performance. The limitation of the direct reduction process is the quality of coke or coal as they provide limited reaction conditions with low productivity.
3.1 Reduction of iron ore using coking coal
Iron oxide reduction by carbon is a significant metallurgical process. Theoretically, the reduction might entail either gaseous intermediates or direct reduction [57]. Carbothermic reduction of iron ore using coking coal has been carried out by several researchers and metallurgists using majorly direct reduction, magnetic separation, reduction-smelting, and thermo-mechanical processes. An extensive study on iron ore reduction in their composite aggregates was also reported in the existing literature. Hou et al. [39] investigated a reduction of lump hematite in a carbothermic atmosphere using a coal-based reduction and magnetic separation method. The hematite ore undergoes a pre-reduction process at a maximum temperature of 1000°C in a coal-infested atmosphere before the disintegrated direct reduction of the hematite ore particle is then subjected to magnetic separation to increase the metallic iron concentration of the DRI. Reduction of iron ore in lump or pelletized form in the presence of coking coal entails a solid-solid reaction mechanism where the CO released from the coke directly permeates the surface structure of the iron ore to obtain a metallic iron as a by-product. But the challenge with the use of coking coal in the ironmaking process can be traced to its scarcity and its undoubted tendency to produce DRI with a high carbon deposit. Studies have shown that ferrous metals produced under Ore/coal composite technique using high carbonaceous materials such as coal will give very hard but brittle and ferrous metals as a by-product [18]. Thus, such DRI might exhibit poor mechanical properties which include low malleability, ductility, and machinability tendencies. Man et al. [42] performed a thermogravimetric experiment on an iron/coal composite by direct reduction process at different reduction temperatures in a nitrogen atmosphere. The changes in the mass of the ore/coal composites reveal the extent mechanical degradation occurs based on the different mass loss, heating rate, and heating temperatures. Also, the permeability rate of carbon from the coking coal on the iron ore concentrate determines the quality of DRI expected to be produced from the reduction experiment. Also, an increase in carbon gas penetration around the interstitial surface of the banded iron/coal composite is strongly affected by increasing the heating temperature and heating rate [58]. This may in turn affects the overall performance of the direct reduction experiment process. Among the parameters that are majorly affected by increased carbonaceous activity with the iron/coal composite when the reduction temperature is increased are increased reduction degree, percentage metallic iron, high carbon deposit, and increased iron/coal composite degradation. In contrast, the scarcity of coking coal for the iron and steelmaking process has called for research concerns where metallurgists and material scientists have started looking into non-coking coal, charcoal, biogas, etc. Another viable alternative replacement for metallurgical coke is due to its complex method of preparation and negative environmental impact on the end-users.
3.2 Reduction of iron ore using non-coking coal/charcoal
For example, it is predicted that Nigeria has over 2 billion metric tons of coal reserves [59]. Although it once possessed a few small coal-fired power stations none of them are still in use today. The government plans to use coal to generate 30% of the nation’s electricity, according to repeated statements from the mining ministry. Additionally, some observers think non-coking coal could help Nigeria mitigate its energy crisis [60]. For instance, in some coal-rich states in the north-central region of Nigeria, a 1200-megawatt (MW) coal-fired power station was envisioned [61]. The project has been frequently put on hold due to financial issues [62]. Later, two more coal plants were planned, although no plans have been made public since the government announced its intention to build them in 2011 [63]. Thus, the socio-economic and manufacturing shortcomings ravaging the usability and accessibility of coking coal in the iron and steel industry have led to the need to seek other reductants as alternative energy sources (i.e. non coking coal, charcoal, biogas, etc.) which can be used in the production of DRI in convectional iron and steelmaking process. Several works of literature have attempted to research the feasibility and viability of these reducing agents using different ironmaking production routes as tremendous processes have been reported about some of the DRI produced when compared to those of the DRI produced in the metallurgical industry [64, 65]. These have shown to have similar and even better quality in terms of their physical, metallurgical, and mineralogical properties.
Although lump iron ore is less expensive than the pelletized version, they have historically been limitations on its usage in blast furnaces due to long-standing worries about its deterioration and high-temperature characteristics. As a result of high-grade iron ore depletion in supplies, gangue minerals are also becoming more prevalent in iron ore fines, which have caused a significant change in their sinter chemistry, particularly in terms of alumina content and basicity.
Lu et al. [66] performed a direct reduction and magnetic separation process on lump hematite ore using lignite as a reductant. Lignite is low-grade non-coking coal of low ash and higher carbon content than bitumen. The reduction roasting was done at 1200°C with 35% lignite on low-graded lump hematite ore with a particle size range of (20–50 mm) at a reduction time of (60–300 mins), the reduced iron ore was ground into a two-stage concentrate (20/30 mins) of 90% theoretical Iron (TFe) and 89.3% iron recovery. The effect of reduction time, roasting temperature, particle dosage, and grindability on the metallization degree, iron recovery, and microstructure phase change on the reduced hematite ore were investigated. Ogbezode et al. [29] examined the kinetic reduction behavior of goethite-hematite ore using wood charcoal in an activated carbon furnace. The DRI produced was achieved through the non-contact reduction of goethite-hematite ore acquired as run-off mines from selected ore depots. The study aims to investigate the behavioral pattern of iron ore in the CO/CO2 atmosphere from wood charcoal. The kinetic model employed was the shrinking core model. The model attests to the fact that goethite ore can be reduced under the CO/CO2 atmosphere where diffusion through the ash layer is the rate-controlling resistance of the reaction kinetic model. The microstructure phase showed a trace amount of carbon deposits on the direct reduced iron compared to using coking coal as carbon-bearing materials regardless of reduction temperature and reaction residence time [30]. From existing literature, the use of non-coking or charcoal as an alternative energy source instead of metallurgical coke in the production of direct reduced iron is targeted toward reducing energy consumption, decreasing carbon deposition, and eliminating complex procedures that accompany the conventional iron sintering processes [32, 67]. Also, the high energy demand and complex procedures involved in the pelletization process of iron ore, coupled with the scarcity of coking coal have made metallurgical scientists begin research into the direct use of iron ore in lump form and the use of non-coking coal or charcoal as reductant [20]. Production of DRI using iron ore in lump form may seem to be a viable alternative for already beneficiated iron ore provided the base material is of high-grade quality. Furthermore, the use of low-grade iron ore could better be encouraged provided a more efficient coal-based direct reduction DR process is employed. The treatment of low-grade iron ore material may hold promise for the coal-based DR process in the future. Therefore, solid carbon or gaseous carbon monoxide CO and hydrogen gas H2 are needed as reducing agents in commercial techniques used in the removal of oxygen from iron ore. The latter can also be obtained naturally through carbon gasification [68]. Thus, it is advisable to take into account the usage of non-coking coal, first directly and then as a gasifying agent to produce the reductant components of CO and H2 gases. Agrawal et al. [49] performed carbothermal reduction on low-grade magnetite ore in a microwave at an irradiation temperature above 600°C using coal and charcoal. There are two different types of composites were prepared which are made of iron ore fines and charcoal, respectively. Magnetite reduction with coal was discovered to be lean, resulting in poorer metallization; yet, the concentrate’s increased iron-oxide content and also increases the iron extraction in terms of performance as compared to charcoal [69]. Regardless of thermal conditions, iron ore is reduced under the same heating rate, and coal has a lower reduction rate compared to charcoal.
The optimum performance of the reduction roasting of low-graded iron ore using non-coking coal should better reveal high theoretical percentage iron (TFe) of good magnetic concentrate which can produce metallic iron with higher purity, which means that a high-temperature reduction process should be encouraged in such cases. Thus, to effectively perform a coal-based reduction on low-grade iron ore using non-coking coal, a high reduction temperature, increased roasting time, and coal with high carbon content, low moisture, and low ash content should be used in other to achieve a sustainable reduction process. The carbon-iron ore dosage must be understood to ascertain the reduction behavioral pattern and phase transformation of the various gangue components present within the metal matrix of the coal-based reduced iron. The non-availability of solid carbon-bearing materials has triggered the need for metallurgical experts to begin to harness a more efficient approach to the production of molten iron in its pure form due to the presence of unwanted substances such as sulfur, phosphorus, lead, etc. that might have accompanied such DRI products. This concern among others does initiate the need to commence further research into the gas-solid reduction of iron ore or iron oxides. Consequently, further studies into the indirect reduction process of iron ore are majorly carried out on a laboratory scale due to their high production cost, purity of DRI product, environmental friendliness, and less hazardous process where its major limitation remains its high cost of production at an industrial scale.
4. Gaseous-solid reduction in iron ore processing
Many researchers have postulated different ways of producing quality iron using gaseous reductants such as carbonmonoxide [14], Hydrogen [70], Methane [71], biogas [72], etc. This is a method of producing direct reduced iron DRI using gaseous reductants as carbon-bearing material. To comprehend the use of gaseous carbonaceous materials as iron oxide reductants, the different phase changes within the macro and microstructure of the processed iron oxides must be known. A schematic illustration of the ironmaking process by direct reduction method using gaseous reductants is depicted in Figure 9. The effect of thermal decomposition, reduction time, and reaction kinetics surrounding the type of reduction mechanism and metallurgical processes used in the production of such metallic iron must be ascertained.
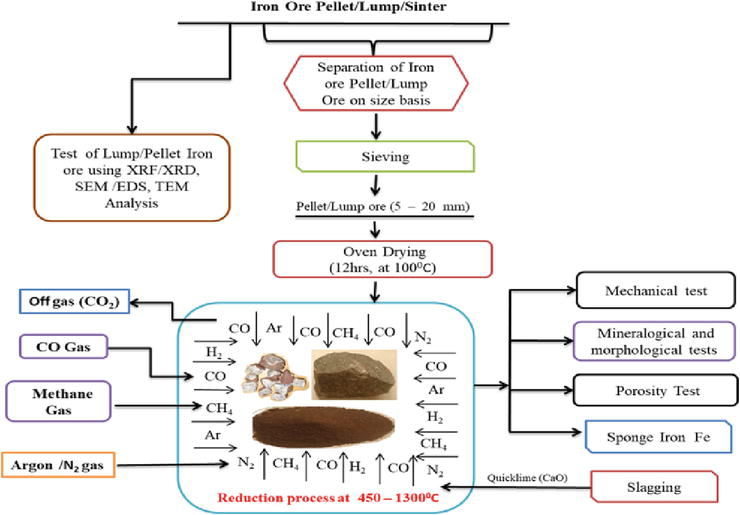
Figure 9.
Schematic flow chart of the reduction process of iron ore using gaseous reductants.
4.1 Reduction of iron ore by hydrogen and carbon-monoxide gas
Due to the adverse effect of carbon-infested gases on our immediate environment, the manufacturing and metallurgical industries produce crude steel of over 1.7 billion tons, while almost the total production of up to 75.1% is carried out using coke-injected blast furnaces [73]. It is no longer news that coking coals can serve as an excellent reducing agent and high energy source for iron and steel production. But the under-utilized coke is produced as carbon monoxide gas which has an adverse climatic effect on our immediate environment [8]. The carbon monoxide gas may also lead to ozone depletion, the greenhouse effect, and global warming at large. These and many more sponsored the initiative for the use of other sources of carbon-bearing substances and gases as alternative energy sources instead of coke for iron ore reduction. This implies that the use of hydrogen, methane, fossil fuels and biomass is fast replacing coke, especially in metallurgical operations. Lately, hydrogen reduction has proven to be a viable alternative in the ironmaking process compared to the conventional carbothermic reduction processes. Li et al. [70] conducted a direct reduction process of iron ore using hydrogen gas (H2). The hydrogen was formed by the catalytic breakdown of ammonia and methane as a way to mitigate CO2 emissions. The by-product hydrogen gas is passed into the fluidized bed reactor containing the sets of cohesive hematite ore particles at a reduction temperature of 500°C and above. Several works of literature [37, 40, 41] have investigated the energetic and environmental essence of the hydrogen-based iron production process. The literature further reiterated that H2−based direct reduction of iron ore possesses a carbon-free iron product with a prospective reduction of harmful gas emissions regardless of the kind of reduction or smelting reactor used [74]. Since the formation of iron-carbide is majorly associated with iron oxide reduced with carbon-monoxide, the use of hydrogen gas as the alternative reductant in the ironmaking process cannot be overemphasized. Another attribute of H2-based direct reduction of iron ore is the physical characteristics and reduction kinetics mechanism associated with such an iron reduction process. These characteristics are reduction temperature, reaction control mechanism, particle size, reduction gas penetration rate, mineral phases, and morphological dependence [52, 75]. Consequently, the behavioral changes in the mineralogical phases of iron ore sinter due to the effect of hydrogen gas concentration were investigated by Xing et al. [76]. The study of mineral phases on iron ore sinter reduction in hydrogen atmosphere has proven to mitigate the effect of CO2 emission in the ironmaking process. Also, whisker growth in direct reduced iron can be attributed to the presence of sulfur present in the reductant coals. As the presence of sulfur in the carbonaceous atmosphere during the ironmaking process possesses the tendency to initiate catastrophic swelling in the iron ore-carbon monoxide atmosphere which may produce a large number of iron whiskers. The presence of sulfur in the CO/CO2-infested iron ore reduction atmosphere is triggered by the nucleation effect of sulfide inhibits gases, resulting in whisker growth. To eliminate the occurrence of whiskers on iron oxide surfaces, hydrogen gas should be used as an alternative reducing agent in the ironmaking process in the presence of argon gas. Moreover, the use of hydrogen naturally eliminated sulfur and also speed up the reduction rate of the base material. Carbothermal reduction of iron oxide is less pure because of the high chemical activity of carbon monoxide and its inability to exist longer in its free state. CO’s ability to exist as a transition metal entails it can also exist in carbide forms. Thus, to mitigate the aftermath effect of challenges caused by the use of CO/CO2 on iron oxide during reduction, the use of hydrogen [77], water gas [78], and hydrocarbon [79] have been encouraged in recent works of literature [18, 19, 47, 80]. Therefore, hydrogen usage in the iron and steelmaking industry remains a prominent way to curtail the menace of the greenhouse effect, energy loss, and global warming in our immediate environment.
4.2 Reduction by methane gas and other hydrocarbons
The reduction of iron ore by carbon monoxide can produce carbondioxide which is utilized by the ecosystems for anaerobic respiration and photosynthetic operations [80]. Consequentially, the oxidation of methane gas and other hydrocarbons does produce water gas (CO and H2) which is charged into a fluidized bed reactor for iron and steel production. Nasr et al. [71] used the chemical looping combustion method to investigate the reduction kinetics of iron ore in a methane atmosphere. The increase in CO2 concentration in the atmosphere has attracted considerable interest in the metallurgical industry. Thus, the use of methane gas as an alternative source of carbon-bearing material has been uninitialized as a promising concept for capturing CO2 in the atmosphere and for power generation via the chemical looping combustion (CLC) method. The reduction of iron ore in the methane atmosphere may not follow the usual stepwise order (i.e. it is either the magnetite stage or the wustite state is being bypassed). This implies the rate of reaction will be faster in the iron oxide/methane reduction reaction as the burning of methane gas in the absence of air produces a chemical loop of CO and H2 which makes the reduction reaction of iron oxide to iron proceed much faster into completion compared to when using the individual reducing gas (i.e. CO and H2). Thus, the reduction of hematite (α-Fe2O3) and maghemite (γ-Fe2O3) in the CH4 atmosphere at standard temperature and pressure in the absence of air will produce iron in the following chronological order Fe2O3 → Fe3O4 → Fe and Fe2O3 → FeO → Fe respectively [35, 66]. This reduction method provides good support performance, increases mechanical strength, and provides attrition resistance for metallic iron produced under environmentally safe conditions and economically viable. Thus, for increased productivity and a high reduction rate, the direct reduction of iron ore with methane does have intense catalytic nature that can be used in the powder metallurgical process.
4.3 Reduction in biomass gas
Biomass is a common solid fuel, and its pyrolysis products, with highly reductive characteristics which occur in solid, liquid, and gaseous states [81]. The quest for coal substitutes in the iron ore reduction process has attracted considerable interest, as the use of pyrolysis products can be viewed as an alternative reductant. This need has its implication to acquire iron ore reduction by metallurgical industries which possess good quality carbon-bearing properties with reduction characteristics, easy renewability, lower greenhouse effect, and fewer energy-saving challenges. The research on pine saw dust usage, as a reductant for pure Fe2O3 particles was investigated by Liu et al. [72]. Biomass pyrolysis of pure Fe2O3 was conducted on pine sawdust at a mass ratio of 3:2 at a calcination time of 15mins. The mixture was fired at a reduction temperature range of 350 – 550°C under a nitrogen-regulated atmosphere. Iron oxide reduced with biomass sawdust can produce pollution-free pure metallic iron. Few research works have attempted to study the use of biomass in the ironmaking process. Gan et al. [82] examined the pollutant effect on sintered iron ore reduced using biomass fuel as a reductant. Luo et al. [83] uses biomass syngas to perform direct reduction on hematite-biomas briquette. The briquette powdered ore-biomass was heated at a reduction temperature range of 450 to 900°C. Strezov, et al. [84, 85], performed iron ore reduction runs using biomass sawdust. The iron was reduced from 30% sawdust to predominantly metallic iron at a reduction temperature above 600°C. The reduction of the iron ore-sawdust mixture begins precisely at 670°C and ended at 1200°C. The implication of using biomass in the ironmaking process is that the process is less energy-consuming, sulfur free, and environmentally friendly without causing the greenhouse effect.
Furthermore, due to the vast utilization capacity of the different iron processing methods in modern-day iron and steel-making technology, it becomes imperative and advantageous to enumerate the basic challenges and limitations peculiar to convectional iron and steel production routes by proferring meaningful solutions and mapping-out holistic future perspectives to issue surrounding extraction process of pure metallic iron. Thus, this study suggests the need to employ a more pragmatic metallurgical extraction approach in the production routes for iron processing with lower production costs and minimal energy requirements [86, 87]. This can also be attributed to the ability to utilize other alternative energy sources instead of the use of metallurgical coke as a reductant. This will lower the primary operation cost, energy consumption, and elimination of operational tendencies for metallurgical coke, and pre-reduction processes (i.e. beneficiation, pelletization, recycling, etc.) may no longer be necessary, the SR process utilizes direct fines and sinters as feeds, better energy conservative process with rich off gas used for power generation, better control of production process parameters (temperature, gas flow rate, reduction rate, etc.). Faster reduction rate and quick iron recovery are certain, as good thermo-chemical configuration and flexibility in hot metal-slag separation, faster reaction kinetics due to high smelting intensity and productivity, etc. [13, 88]. In contrast, the SR process also has some limitations despite its economic viability and high productivity tendencies. This includes high oxygen and power consumption requiring mandatory ore fine pre-reduction process and the need to meet up with required energy for a highly efficient post-combustion process.
5. Future research perspective
In the quest to improve the quality of iron produced across the globe, so many works have been done through the development of various ironmaking and steelmaking techniques. Many ideas have been developed on how to improve the various metallurgical operations governing the extraction process of iron and steel research all over the world. These iron extraction processes are mainly categorized into the blast furnace, direct reduction, indirect reduction, and reduction-smelting processes. Metallurgical factors such as reduction agent types, quality of iron oxide, energy sources and analysis, cost of production, etc. are scarcely considered in the works of literature. Thus, it is expedient that future research perspectives should consider the aforementioned factors affecting the overall metallurgical extraction process in iron and steel production before active application in extraction technique. In addition, the influence of foreign bodies in the metal matrix of iron metals produced by the different extraction processes should be checked and excess silicate and aluminate with the banded microstructure of iron oxide metal matrix will require the use of the excessive fluxing agent, increased energy demand during the iron smelting process. Thus, further studies on the effect of gangue elements on the iron metal quality in convectional iron and steelmaking processes should be further investigated.
6. Conclusion
The fast depletion of metallurgical coke in the present era iron and steelmaking making process remains an eye-opener for metallurgical experts across the globe. This challenge has reiterated the need to seek other alternative energy sources for the iron and steelmaking process with viable production routes. Due to economic implications, complex metallurgical operations, high energy demand, and time-consuming tendencies that accompany the conventional blast furnace/blast oxygen furnace operation and direct reduction route. This study provides an extensive overview of selected iron and steelmaking processes with special emphasis on the various alternative energy sources that are used instead of conventional metallurgical coke. Thus, the study concludes as follows:
Apart from the traditional method of iron production using the blast furnace process, the direct reduction process, indirect reduction process, and smelting-reduction process remains the most recent trend used in present-era ironmaking technology.
The use of gaseous reductants (i.e. CO, H2, CH4, etc.) remains a viable alternative energy source provided they can be acquired at a cheaper rate as the usage can be unsustainable when applied in iron and steelmaking processes at an industrial scale.
The operational capabilities of the conventional direct smelting process can be improved upon when modified with a post-reduction process within its ambiance provided the use of energy saving approach is encouraged.
The non-contact direct reduction technique has shown its viability for decarburization of direct reduct iron with minimal energy requirement and it has shown to be cost-effective.
The smelting-reduction technique has an advantage over direct reduction and indirect reduction processes as it holds the capacity to utilize many available energy sources which makes it more time-saving, less energy-consuming, and operationally efficient.
The use of gaseous reductants in the smelting-reduction process of iron oxide remains a more promising approach in the production of pure molten iron, free from unwanted impurities within the phase microstructure of the by-product.
Acknowledgments
This work was supported by the Petroleum Technology Development Fund, Nigeria. Grant Number: PTDF/ED/LSS/PhD/JEO/0278/19, 2019.
References
- 1.
Ahmed H. New trends in the application of carbon-bearing materials in blast furnace iron-making. Minerals. 2018; 8 (12):561. DOI: 10.3390/min8120561 - 2.
Abdus-Salam N, Ugbe FA, Funtua MA. Characterization of synthesized goethite and natural goethite sourced from Itakpe in north central, Nigeria. Chemistry Search Journal. 2018, 2018; 9 (2):24-32 - 3.
Abdel Halim KS. Isothermal reduction behavior of Fe2O3/MnO composite materials with solid carbon. Materials Science and Engineering: A. 2007; 452-453 :15-22. DOI: 10.1016/j.msea.2006.12.126 - 4.
Alamsari B, Torii S, Trianto A, Bindar Y. Heat and mass transfer in reduction zone of sponge iron reactor. Journal International Scholarly Research Network, ISRN Mechanical Engineering. 2011:1-12. DOI: 10.5402/2011/324659 - 5.
Siddiqi H, Chandaliya VK, Suresh A, Dash PS, Meikap BC. A scale-up approach to produce highly reactive iron ore catalyzed coke for blast furnace operation. Mineral Processing and Extractive Metallurgy Review. 2019; 41 :1-11. DOI: 10.1080/08827508.2019.1666124 - 6.
Babich A, Mousa EA, Senk D. Reduction behavior of iron ore pellets with simulated coke oven gas and natural gas. Steel Research International. 2013; 84 (11):1085-1097. DOI: 10.1002/srin.201200333 - 7.
Bahgat M, Abdel Halim KS, El-Kelesh HA, Nasr MI. Blast furnace operating conditions manipulation for reducing coke consumption and CO2 emission. Steel Research International. 2012; 83 (7):686-694. DOI: 10.1002/srin.201200001 - 8.
Angalakuditi VB, Bhadravathi P, Gujare R, Ayyappan G, Singh LR. Mineralogical aspects of reducing lump iron ore, pellets, and sinter with hydrogen. Metallurgical and Materials Transactions B. 2022; 53 :1036-1065. DOI: 10.1007/s11663-022-02442-4 - 9.
Arisanti R, Yusuf M, Faizal M. Study of the effect of proximate, ultimate, and calorific value analysis on methane gas emission (CH4) on combustion of coal for sustainable environment. Science and Technology Indonesia. 2018; 3 (2):100-106. DOI: 10.26554/sti.2018.3.2.100-106 - 10.
Romenets VA, Usachev AB, Balasanov AV, Lekherzak VE. The role of coal in the Romelt process for the liquid-phase reduction of iron. Metallurgist. 2001; 45 (3/4):101-105. DOI: 10.1023/a:1010555923457 - 11.
Roy S, Das A. Characterization and processing of low-grade iron ore slime from the Jilling area of India. Mineral Processing and Extractive Metallurgy Review. 2008; 29 (3):213-231. DOI: 10.1080/08827500801997886 - 12.
Sah R, Dutta SK. Smelting reduction of iron ore-coal composite pellets. Steel Research International. 2010; 81 (6):426-433. DOI: 10.1002/srin.200900113 - 13.
Shekhawat D, Kukshal V, Banerjee MK, Patnaik A. Study the reduction of mill scale with lean grade coal through RI-RDI. IOP Conference Series: Materials Science and Engineering. 2021; 1017 :1-13. DOI: 10.1088/1757-899X/1017/1/012037 - 14.
Sharma MK, Solanki V, Roy GG, Sen PK. Study of reduction behaviour of prefabricated iron ore–graphite/coal composite pellets in rotary hearth furnace. Journal of Ironmaking and Steelmaking. 2013; 40 :590-597. DOI: 10.1179/1743281212Y.0000000086 - 15.
Wan X, Shen L, Jokilaakso A, Eriç H, Taskinen P. Experimental approach to matte–slag reactions in the flash smelting process. Mineral Processing and Extractive Metallurgy Review. 2020; 42 :1-11. DOI: 10.1080/08827508.2020.1737801 - 16.
Wang H, Sohn HY. Effects of firing and reduction conditions on swelling and iron whisker formation during the reduction of iron oxide compact. ISIJ International. 2011; 51 (6):906-912. DOI: 10.2355/isijinternational.51.906 - 17.
Wang HT, Sohn HY. Effect of CaO and SiO2 on swelling and iron whisker formation during reduction of iron oxide compact. Ironmaking & Steelmaking. 2011; 38 (6):447-452. DOI: 10.1179/1743281211y.0000000022 - 18.
Mousa EA, Babich A, Senk D. Utilization of coke oven gas and converter gas in the Direct Reduction of lump iron ore. Metallurgical and Materials Transactions B. 2013; 45 (2):617-628. DOI: 10.1007/s11663-013-9978-6 - 19.
Qie J, Zhang Y, Wang J, Zhang X, Fu T, Cui K. Reduction and melting behavior of pre-reduction metallized carbon-bearing pellets in iron Bath smelting reduction. Journal of Materials. 2022; 74 :1-10. DOI: 10.1007/s11837-021-05104-z - 20.
Liu Z, Bi X, Gao Z, Liu W. Carbothermal reduction of iron ore in its concentrate-agricultural waste pellets. Advances in Materials Science and Engineering. 2018; 2018 :1-6. DOI: 10.1155/2018/2138268 - 21.
El-Geassy AA, Nasr MI, El-Raghy SM, Hammam AA. Comparative studies on isothermal and non-isothermal reduction of Haematite in carbon monoxide atmosphere. Ironmaking & Steelmaking. 2019; 47 :1-10. DOI: 10.1080/03019233.2019.1646564 - 22.
Donskoi E, McElwain DL, Wibberley LJ. Estimation and modelling of parameters for direct reduction in iron ore/coal composites: Part II. Kinetic parameters. Journal of Metallurgy and Materials Transaction B. 2003; 34 :255-266. DOI: 10.1007/s11663-003-0012-2 - 23.
Donskoi E, Olivares RI, McElwain DL, Wibberley LJ. Experimental study of coal based direct reduction in iron/coal composite pellets in a one layer bed under non-isothermal asymmetric heating. Journal of Ironmaking and Steelmaking. 2006; 33 :24-28. DOI: 10.1179/174328106X80064 - 24.
Hasanbeigi A, Arens M, Price L. Alternative emerging ironmaking technologies for energy efficiency and carbon dioxide emissions reduction: A technical review. Renewable and Sustainable Energy Reviews. 2014; 33 :645-658. DOI: 10.1016/j.rser.2014.02.031 - 25.
Ramakgala C, Danha G. A review of ironmaking by direct reduction processes: Quality requirements and sustainability. Procedia Manufacturing. 2019; 35 :242-245. DOI: 10.1016/j.promfg.2019.05.034 - 26.
Folorunso DO, Olubambi P, Borode JO. Characterization and qualitative analysis of some Nigerian clay deposits for Rafractory applications. IOSR Journal of Applied Chemistry (IOSR-JAC). 2014; 7 (9):40-47. DOI: 10.9790/5736-7914047 - 27.
Anameric B, Kawatra SK. Direct iron smelting reduction processes, mineral processing, and extractive metallurgy review: An. International Journal. 2008; 30 (1):1-51. DOI: 10.1080/08827500802043490 - 28.
Gojic M, KoÞuh S. Development of direct reduction processes and smelting reduction processes for the steel production. Journal of Chemists and Chemical Engineers. 2022; 55 (1):1-10 - 29.
Ogbezode JE, Oluwole OO. Reduction kinetics behavior of goethite iron ore in the CO/CO2 atmosphere from wood charcoal. International Journal of Emerging Engineering Research and Technology (IJEERT). 2019; 7 (4):11-21 - 30.
Ogbezode J, Ajide O, Ofi O, Oluwole OO. Determination of the reaction rate controlling resistance of goethite iron ore reduction using CO/CO2 gases from wood charcoal. Material Proceedings, MPDI. 2021; 3 (1):27. DOI: 10.3390/IEC2M-09373 - 31.
Ogbezode J, Ajide O, Ofi O, Oluwole O. An overview of the reduction-smelting process of iron oxides in modern-day ironmaking technology. Research and Development in Material Science (RDMS). 2022; 17 (4):1992-1994. DOI: 10.31031/RDMS.2022.17.000918 - 32.
Muwanguzi AJB, Karasev AV, Byaruhanga JK, Jönsson PG. Characterization of chemical composition and microstructure of natural iron ore from Muko deposits. ISRN Materials Science. 2012:1-9. DOI: 10.5402/2012/174803 - 33.
Zhu D, Luo Y, Pan J, Zhou X. Characterization of semi-coke generated by coal-based direct reduction process of siderite. Journal of Central South University. 2015; 22 (8):2914-2921. DOI: 10.1007/s11771-015-2826-x - 34.
Huitu K, Helle M, Helle H, Kekkonen M, Saxén H. Optimization of Midrex direct reduced iron use in ore-based steelmaking. Steel Research International. 2014; 86 (5):456-465. DOI: 10.1002/srin.201400091 - 35.
Heidari A, Niknahad N, Iljana M, Fabritius TA. Review on the kinetics of iron ore reduction by hydrogen. Materials. 2021; 14 :7540. DOI: 10.3390/ma14247540 - 36.
Li K, Wen N, Zhu M, Zheng M, Yuan L. Iron extraction from Oolitic iron ore by a deep reduction process. Journal of Iron and Steel Research International. 2011; 18 (8):9-13. DOI: 10.1016/s1006-706x(11)60096-4 - 37.
Bhaskar A, Assadi M, Nikpey SH. Decarbonization of the iron and steel industry with direct reduction of iron ore with green hydrogen. Energies. 2020; 13 (3):758. DOI: 10.3390/en13030758 - 38.
Gonoring TB, Franco AR Jr, Vieira EA, Nascimento RC. Kinetic analysis of the reduction of hematite fines by cold hydrogen plasma. Journal of Materials Research and Technology. 2022; 20 :2173-2187. DOI: 10.1016/j.jmrt.2022.07.174 - 39.
Hou B, Zhang H, Li H, Zhu Q. Study on kinetics of iron oxide reduction by hydrogen. Chinese Journal of Chemical Engineering. 2012; 20 (1):10-17. DOI: 10.1016/s1004-9541(12)60357-7 - 40.
Du W, Yang S, Pan F, Shangguan J, Lu J, Liu S, et al. Hydrogen reduction of hematite ore fines to magnetite ore fines at low temperatures. Journal of Chemistry. 2017:1-11. DOI: 10.1155/2017/1919720 - 41.
Vogl V, Åhman M, Nilsson LJ. Assessment of hydrogen direct reduction for fossil-free steelmaking. Journal of Cleaner Production. 2018; 203 :736-745. DOI: 10.1016/j.jclepro.2018.08.279 - 42.
Ma Y, Souza FIR, Zhang X, Nandy S, Barriobero-Villa P, Requena G, et al. Hydrogen-based direct reduction of iron oxide at 700°C: Heterogeneity at pellet and microstructure scales. International Journal of Minerals, Metallurgy, and Materials. 2022; 29 (10):1901-1907. DOI: 10.1007/s12613-022-2440-5 - 43.
Naseri Seftejani M, Schenk J, Zarl MA. Reduction of Haematite using hydrogen thermal plasma. Materials. 2019; 12 (10):1608. DOI: 10.3390/ma12101608 - 44.
Naseri Seftejani M, Schenk J. Thermodynamics of liquid iron ore reduction by hydrogen thermal plasma. Metals. 2018; 8 (12):1051. DOI: 10.3390/met8121051 - 45.
Naseri Seftejani M, Schenk J, Spreitzer D, Andreas ZM. Slag formation during reduction of iron oxide using hydrogen plasma smelting reduction. Materials. 2020; 13 (4):935. DOI: 10.3390/ma13040935 - 46.
Fowkes N. The reduction of iron ore pellets used in the FASTMET process. IMA Journal of Management Mathematics. 1999; 10 (1):27-39. DOI: 10.1093/imaman/10.1.27 - 47.
Youling L, Huibin L, Hua W, Shan Q, Jianhang H, Yali H, et al. Smelting potential of HIsmelt Technology for High-Phosphorus Iron ore and Ilmenite. In: 2011 International Conference on Computer Distributed Control and Intelligent Environmental Monitoring. IEEE Computer Society. 2011. DOI: 10.1109/cdciem.2011.353 - 48.
Shi B, Zhu D, Pan J, Hu B, Wang Z. Reducing process of sinter in COREX shaft furnace and influence of sinter proportion on reduction properties of composite burden. Journal of Central South University. 2021; 28 (3):690-698. DOI: 10.1007/s11771-021-4638-5 - 49.
Agrawal S, Dhawan N. Microwave Carbothermic reduction of low-grade iron ore. Metallurgical and Materials Transactions B. 2020; 51 :1576-1586. DOI: 10.1007/s11663-020-01883-z - 50.
Murakami T, Shinomiya H, Maruoka D, Kasai E. Effect of types of carbonaceous material and CaO addition on reduction behavior of pre-reduced iron ore–carbon composite. ISIJ International. 2019; 59 (6):1011-1017. DOI: 10.2355/isijinternational.isijint-2018-725 - 51.
Khasraw D, Spooner S, Hage H, Meijer K, Li Z. Devolatilisation characteristics of coal and biomass with respect to temperature and heating rate for HIsarna alternative ironmaking process. Fuel. 2021; 284 :119101. DOI: 10.1016/j.fuel.2020.119101 - 52.
Zhang L, Zhu Y, Yin W, Guo B, Rao F, Ku J. Isothermal coal-based reduction kinetics of Fayalite in copper slag. ACS Omega. 2020; 5 (15):8605-8612. DOI: 10.1021/acsomega.9b04497 - 53.
Li H, Pinson DJ, Zulli P, Lu L, Longbottom RJ, Chew SJ, et al. Interaction between mineral phases in a hematite iron ore and fluxing materials during sintering. Metallurgical and Materials Transactions B. 2020; 52 :267-281. DOI: 10.1007/s11663-020-02010-8 - 54.
Kumar M, Baghel H, Patel KS. Reduction and swelling of fired hematite iron ore pellets by non-coking coal fines for application in sponge ironmaking. Mineral Processing and Extractive Metallurgy Review: An International Journal. 2013; 34 (4):249-267. DOI: 10.1080/08827508.2012.656776 - 55.
Kumar M, Patel SK. Characteristics of Indian non-coking coals and iron ore reduction by their chars for directly reduced iron production. Mineral Processing and Extractive Metallurgy Review. 2008; 29 (3):258-273. DOI: 10.1080/08827500801997902 - 56.
Pal J, Arunkumar C, Rajshekhar Y, Das G, Goswami MC, Venugopalan T. Development on iron ore Pelletization using calcined lime and MgO combined flux replacing limestone and bentonite. ISIJ International. 2014; 54 (10):2169-2178. DOI: 10.2355/isijinternational.54.2169 - 57.
Haque R, Ray HS. Role of ore/carbon contact and a direct reduction in the reduction of iron oxide by carbon. Metallurgical and Materials Transactions B. 1995; 26 (2):400-401. DOI: 10.1007/bf02660982 - 58.
Fortini OM, Fruehan RJ. Rate of reduction of ore-carbon composites: Part I. determination of intrinsic rate constants. Metallurgical and Materials Transactions B. 2005; 36 (6):865-872. DOI: 10.1007/s11663-005-0088-y - 59.
Onyedika G, Nwoko C, Oguarah A, Ogwuegbu M. Comparative kinetics of iron ore dissolution in aqueous HCl-HNO3 system. Journal of Minerals and Materials Characterization and Engineering. 2013; 1 :153-159. DOI: 10.4236/jmmce.2013.14026 - 60.
Odokuma-Alonge O. A geochemical appraisal of some marble physiques in Ubo area and environs, southwestern Nigeria. Journal of Applied Sciences and Environmental Management. 2019; 23 (6):1189. DOI: 10.4314/jasem.v23i6.29 - 61.
Jimoh OA, Ariffin KS, Hussin HB, Habeeb AA. Characterization and assessment of Okpella Metacarbonate deposit in Nigeria. Carbonates and Evaporites. 2016; 32 (4):513-524. DOI: 10.1007/s13146-016-0308-3 - 62.
Oluwatoyin OA, Olusola AO. Lithological features and chemical characterization of metamorphosed carbonate rocks in Igue, southwestern, Nigeria. Journal of Geology and Mining Research. 2021; 13 (1):11-20. DOI: 10.5897/JGMR2020.0349 - 63.
Olayebi OO. Steelmaking experience in the use of Nigerian in ore at the Delta steel company, Nigeria. Journal of Chemical Engineering and Materials Science. 2014; 5 (5):47-62. DOI: 10.5897/JCEMS2014.0166 - 64.
Omang BO, Kudamnya EA, Owolabi AO, Odey J, Aniwetalu EU, Ako TA. Characterization of kaolin deposits in Okpella and environs southern Nigeria. International Journal of Geosciences. 2019; 10 :317-327. DOI: 10.4236/ijg.2019.103018 - 65.
Omole SO, Oyetunji A, Alaneme KK, Olubambi PA. Structural characterization and mechanical properties of pearlite-enhanced micro-alloyed ductile irons. Journal of King University-Engineering Sciences. 2018; 32 (3):205-210. DOI: 10.1016/j.jksues.2018.11.008 - 66.
Lu C, Li K, Wang H, Zhu X, Wei Y, Zheng M, et al. Chemical looping reforming of methane using magnetite as oxygen carrier: Structure evolution and reduction kinetics. Applied Energy. 2018; 211 :1-14. DOI: 10.1016/j.apenergy.2017.11.04 - 67.
Subhnit KR, Deepak NND, Swagat SR. Influence of coal petrography on microwave-assisted Carbothermic reduction roasting of banded hematite Jasper ore. Mineral Processing and Extractive Metallurgy Review. 2020; 42, 2020 :1-15. DOI: 10.1080/08827508.2020.1743290 - 68.
Liu G, Strezov V, Lucas JA, Wibberley LJ. Thermal investigations of direct iron ore reduction with coal. Thermochimica Acta. 2004; 410 (1–2):133-140. DOI: 10.1016/s0040-6031(03)00398-8 - 69.
Hammam A, Cao Y, El-Geassy HA, El-Sadek MH, Li Y, Wei H, et al. Non-isothermal reduction kinetics of iron ore fines with carbon-bearing materials. Metals. 2021; 11 :1137. DOI: 10.3390/met11071137 - 70.
Li S, Zhang H, Nie J, Dewil R, Baeyens J, Deng Y. The direct reduction of iron ore with hydrogen. Sustainability. 2021; 13 :8866. DOI: 10.3390/su13168866 - 71.
Nasr S, Plucknett KP. Kinetics of iron ore reduction by methane for chemical looping combustion. Energy & Fuels. 2014; 28 (2):1387-1395. DOI: 10.1021/ef402142q - 72.
Liu X, Zhang H, Li S, Li D, Huang D. Research on reduction of Fe2O3 by biomass sawdust. Journal of Shanghai Jiaotong University (Science). 2017; 22 (3):280-285. DOI: 10.1007/s12204-017-1833-5 - 73.
Speight GJ. Ultimate analysis. In: Handbook of Coal Analysis. John Wiley & Sons; 2015. pp. 144-169. DOI: 10.1002/9781119037699.ch6 - 74.
Htet TT, Yan Z, Spooner S, Degirmenci V, Meijer K, Li Z. Gasification and physical-chemical characteristics of carbonaceous materials in relation to HIsarna ironmaking process. Fuel. 2021; 289 :119890. DOI: 10.1016/j.fuel.2020.119890 - 75.
Bielowicz B. Petrographic characteristics of coal gasification and combustion by-products from high volatile bituminous coal. Energies. 2020; 13 (17):4374. DOI: 10.3390/en13174374 - 76.
Xing L, Qu Y, Wang C, Shao L, Zou Z. Gas–liquid reduction behavior of hematite ore fines in a flash reduction process. Metallurgical and Materials Transactions B. 2022; 51 :1233-1242. DOI: 10.1007/s11663-020-01811-1 - 77.
Pineau A, Kanari N, Gaballah I. Kinetics of reduction of iron oxides by H2. Thermochimica Acta. 2006; 447 (1):89-100. DOI: 10.1016/j.tca.2005.10.004 - 78.
Pavlov AI. Coal gasification as an alternative to natural gas. Steel in Translation. 2009; 39 (6):475-478. DOI: 10.3103/s0967091209060096 - 79.
Palacios P, Toledo M, Cabrera M. 2015. Iron ore reduction by methane partial oxidation in a porous media. International Journal of Hydrogen Energy. 2015; 40 (31):9621-9633. DOI: 10.1016/j.ijhydene.2015.05.058 - 80.
Pavlína P, Simona J. Process engineering in iron production, journal of. Chemical and Process Engineering. 2013; 34 :63-76. DOI: 10.2478/cpe-2013-0006 - 81.
Brázda P, Kohout J, Bezdička P, Kmječ T. α-Fe2O3 versus β-Fe2O3: Controlling the phase of the transformation product of ε-Fe2O3 in the Fe2O3/SiO2 system. Crystal Growth & Design. 2014; 14 (3):1039-1046. DOI: 10.1021/cg4015114 - 82.
Gan M, Fan X, Chen X, Ji Z, Lv W, Wang Y, et al. Reduction of pollutant emission in iron ore sintering process by applying biomass fuels. ISIJ International. 2012; 52 (9):1574-1578. DOI: 10.2355/isijinternational.52.1574 - 83.
Luo S, Yi C, Zhou Y. Direct reduction of mixed biomass-Fe2O3 briquettes using biomass-generated syngas. Renewable Energy. 2011; 36 (12):3332-3336. DOI: 10.1016/j.renene.2011.05.006 - 84.
Strezov V. Iron ore reduction using sawdust: Experimental analysis and kinetic modeling. Renewable Energy. 2006; 31 (12):1892-1905. DOI: 10.1016/j.renene.2005.08.032 - 85.
Tleugabulov SM, Abikov SB, Altybaeva DK, Isupov YD, Tleugabulov BS. Reductive smelting of iron ore. Steel in Translation. 2015; 45 (5):351-355. DOI: 10.3103/s0967091215050162 - 86.
Tleugabulov SM, Eremeeva ZV, Kurmanseitov MB. Carburization and decarburization in iron and steel production. Steel in Translation. 2018; 48 (11):732-736. DOI: 10.3103/s0967091218110116 - 87.
Zhang Y, Gao Q, Zhao J, Li M, Qi Y. Semi-smelting reduction and magnetic separation for the recovery of iron and alumina slag from iron rich bauxite. Minerals. 2019; 9 (4):223. DOI: 10.3390/min9040223 - 88.
Ofoegbu SU. Characterization studies on Agbaja iron ore: A high-phosphorus content ore, SN. Applied Sciences. 2019; 1 :204. DOI: 10.1007/s42452-019-0218-9