The comparison of various properties of PAn-based materials used for MR fluids.
Abstract
Smart fluids are stimuli-responsive materials whose rheological properties can be changed drastically by applying either an external electric or magnetic field strength. Smart fluids are dispersions comprised of dispersed particles in a carrier liquid that transform from liquid-like state to solid-like state within milliseconds reversibly with an application of external field due to the structural chain formation of the dispersed particles. Owing to this outstanding controllable transformation capability, smart fluids are utilized in various potential applications where an electro/magneto-mechanical interface is required, such as dampers, clutches, shock absorbers, robotics, haptic devices, microfluidics, etc. Various kinds of materials have been proposed and used by researchers for applications that require the electrorheological (ER) and magnetorheological (MR) effects. Polyaniline (PAn) is considered a remarkable material as a dispersed phase of ER fluids due to its easy synthesis, low cost, adjustable conductivity through doping/de-doping processes, and excellent environmental stability. PAn is an attractive material in MR fluids as well due to its contribution to the improvement of dispersion stability and protection against corrosion and oxidation of the soft-magnetic particles. In this chapter, the recent advances in the usage of various kinds of PAn-based materials as electric and magnetic field responsive materials and their ER/MR behaviors are summarized.
Keywords
- polyaniline
- polyaniline-based-nanocomposites
- polyaniline-based-hybrid materials
- electrorheological fluids
- magnetorheological fluids
1. Introduction
Electrorheological (ER) and magnetorheological (MR) fluids are stimuli-responsive smart dispersions with rheological properties (yield stress, viscosity, shear modulus, etc.) that are reversible and controllable in a continuous manner with the usage of an externally applied electric field (
For ER fluids, when an
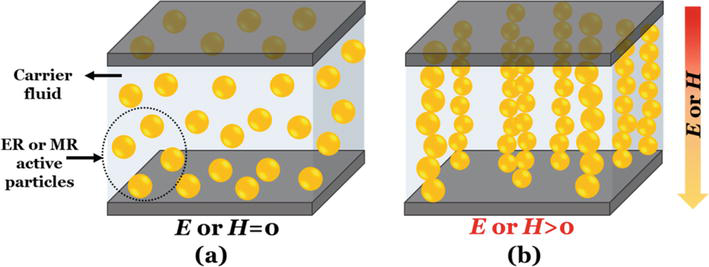
Figure 1.
Schematic representation of the microstructure of ER or MR fluids under off-state (a) and on-state (b) of E or H.
A deeper understanding of the field-induced structuring mechanism may lead us to design and prepare high-performance field responsive fluids. The mechanism of the electric field-induced rheological changes has been studied intensively since the invention of the ER fluids [3]. Several mechanisms or models were proposed previously to explain the ER effect, including fibrillation, electrical double layer, water/surfactant bridge, polarization, conduction, and dielectric loss model [4]. On the other hand, particle magnetization model is the most acceptable mechanism to account for the magnetic field-induced structuring in MR fluids. According to the particle magnetization model, the MR effect is ascribed to the magnetic permeability mismatch between the dispersed particles and continuous phases [2]. There are several critical key factors that influence the behavior of the ER fluids. Thus, it is difficult to develop desired high-performance ER fluids in consideration of all variables such as dispersed particle size, shape and overall morphology, particle conductivity and dielectric properties, particle surface properties, particle volume fraction of the ER fluid, the
As a dispersed phase, a wide range of ER or MR active materials are developed to eliminate the impediments that limit the use of stimuli-responsive fluids in the literature. Various polarizable particles ranging from ceramics to polymers have been applied as the dispersed phase of ER fluids, including SiO2, TiO2, corn starch, aluminosilicate, carbonaceous particles, conducting polymers, etc. [1]. In addition, recent efforts on various hybrid materials with different hierarchical morphologies have been made to improve the dispersion stability and polarizability. As a dispersed phase, Fe3O4, Fe2O3, iron particles, carbonyl iron (CI) particles, iron alloy particles (nickel-iron, cobalt-iron, magnetized stainless steel alloys), and ferrite nanoparticles were used to fabricate MR fluids [7]. MR active particles are desired to have large saturation magnetization and small coercivity value over a wide temperature range, high dispersion, and chemical stability [2]. Although these MR active materials have reasonable mechanical properties, they generally have lack of dispersion stability against settling and poor anticorrosion properties. Thus, surface modification, coating of magnetic particles, or the addition of different additives are usually required. The optimum particle size of dispersed phase is usually in the range of 0.1–10 μm.
Among conductive polymers, PAn has been widely used in the research on ER materials so far due to its possessing excellent chemical and environmental stability and tunable conductivity. Additionally, PAn has been utilized for enhancing the dispersion stability of MR fluids and obtaining dual ER/MR response. In this chapter, the recently developed PAn-based materials as electric and magnetic field responsive materials and their ER/MR behaviors are reviewed.
2. Polyaniline-based ER fluids
As an anhydrous ER active material, PAn and PAn-based materials have been extensively investigated in previous studies due to their adjustable electrical conductivity, easy synthesis, low cost, good environmental, thermal, and chemical stability, and nonabrasive for the device. Various efforts have been made to increase the ER efficiency of PAn, including synthetization of PAn with different morphologies, preparing its derivatives, and combining PAn with various kinds of materials such as inorganic particles or polymers by preparing composites, nanocomposites, core/shell structures, etc. In this section, constituents of PAn-based ER fluids have been reported so far and their general ER characteristics are summarized. The main parameters of PAn-based ER fluids can be found in a brief table in a recent review reported by Kuznetsov et al. [8].
2.1 Development and diversification of polyaniline-based ER fluids
2.1.1 Polyaniline, its derivatives, and copolymers
Among conjugated conductive polymers, PAn has drawn great attention due to its easy and cost-effective production. PAn is synthesized in different morphologies, including fibrillar, tubular, belt-like, porous, hollow structures, etc. PAn exists in different forms in terms of its oxidation state as fully oxidized, half-oxidized, and fully reduced states, namely, pernigraniline, emeraldine base, and leucomeraldine, respectively. For ER application, the level of conductivity of the fabricated PAn is important and depends on the degree of doping, synthesis conditions such as temperature, monomer: oxidatant ratio, presence of surfactant, and other components. If the conductivity of the PAn is not within the proper semiconductive level, dedoping process is generally applied to PAn before preparing ER fluids. The doping process by organic/inorganic acids also impacts the control of wettability of PAn, which plays a role in ER efficiency by influencing the compatibility between solid phase and liquid phase [9, 10].
The doping degree of PAn can be controlled by adjusting the pH of the aqueous solution containing the PAn particles to 9.0 to make it suitable for ER fluids. Additionally, Xie et al. reported the effect of different dedoping methods on ER properties of PAn, namely equilibrium and nonequilibrium. In the reported equilibrium method, the PAn particles were immersed in a diluted aqueous ammonia solution with a certain pH value and stirred for a defined time. On the other hand, in the reported nonequilibrium method, the PAn particles were immersed in a concentrated aqueous ammonia solution for a certain time. Although similar conductivity values were reached for both methods, the greater yield stress value was observed in case of the PAn prepared
Substituted derivatives of PAn are obtained by the introducing of substituted groups on the benzene ring and/or amino N of PAn. The conjugation length and electrochemical behaviors are both affected by the substituent nature and its position on the ring [12]. The PAn derivatives usually show reduced intrinsic conductivities by several orders of magnitude compared to PAn due to steric hindrance of the substituent groups or the disruption of the conjugated backbone or difference in the interchain interactions [13]. Thus, further dedoping process, which is generally required for PAn-based ER fluids, may not be required for PAn derivatives, and the efficiency of the processing of ER fluid can be increased. The most extensively investigated introduced substituents are methyl, ethyl, methoxy, ethoxy, and phenyl groups. There are vast amount of different homopolymers and copolymers have been prepared from substituted anilines for ER application in the literature, including poly(2-methoxyaniline) or poly(
An irregular particulate morphology is generally obtained from chemical oxidative polymerization of aniline and used as ER active material. Additionally, well-defined hollow [26], nanofibrous [27, 28], nanotubular [29], nanorod covered rectangular tubular [30], urchin-like [31], and clip-like [32] shaped PAn have been developed using a variety of synthesis methods, such as template synthesis, rapid mixing polymerization or interface polymerization, chemical oxidation dilute polymerization in an aqueous acidic solution with an anionic surfactant, the
2.1.2 Polyaniline/inorganic hybrid materials
PAn and PAn derivatives have been hybridized with inorganic materials in order to achieve the synergistic contribution of constituent materials, which cannot be provided from one another, to improve ER characteristics and dispersion stability of the fluid and adjust the conductivity in a desired range for ER applications [36]. Several methods have been applied to fabricate PAn/inorganic hybrid materials including
Among the natural clay minerals, inherent 1D nanostructured sepiolite, palygorskite (attapulgite), and halloysite have high aspect ratio and surface charge, large specific surface area, good mechanical strength, outstanding chemical, thermal and dimensional stability, low cost, abundant natural resources, and easy processing and have been hybridized with PAn to utilize their 1D shape effect. In another study, porous zeolite allowed the PAn to fill into microstructural pores resulting in complex nanostructures, leading PAn composite to have better dielectric properties and ER performance due to stronger polarization and fibrillar formation under
2.1.3 Polyaniline/carbon-based nanoparticle composites
Carbon-based nanostructures such as carbon nanoparticles, multi-walled carbon nanotubes (MWCNT), graphene, and graphene oxide (GO) have been utilized for the preparation of ER materials due to their unique and versatile electrical and morphological properties. The carbon-based materials, which stand out with their high conductivity, have been combined with polymers or inorganic compounds in order to reach the appropriate conductivity range for ER studies. These studies were in the form of coating carbon-based material on polymer or inorganic material, or, on the contrary, coating carbon-based material with polymer or inorganic material. Among the carbon-based materials, GO sheets, considered as an oxidation state of graphene, have the most remarkable result since these materials possess relatively reduced electrical conductivity even without any posttreatment and good dispersion stability in carrier fluid due to the large amount of hydroxyl, carboxylic, and epoxide functional groups on its basal planes and edges [83, 84]. PAn has been combined with various carbon-based materials for ER studies, including MWCNT [85], GO [86, 87, 88], graphene [89, 90], and carbon particles [91], in addition, 2D carbonaceous particles were fabricated from annealing of PAn-coated GO sheets in vacuum [92] and core–shell particles of carbonized PAn base was coated with PAn base [93].
In a study, nitrogen-enriched carbonaceous nanotubes with large aspect ratio and tunable conductivity were prepared by heat treatment of PAn nanotubes at elevated temperatures and used as ER active material. Thus, with this approach, to achieve the desired high polarizability and dispersion stability for ER fluids, the high aspect ratio of 1D morphology has been maintained with appropriate electrical conductivity, which is difficult to achieve in highly conductive carbon-based nanostructures. Furthermore, nitrogen-enriched carbonaceous nanotubes displayed better dispersion stability and higher ER performance compared to their granular analogue [29]. In another study, to display the morphology and conductivity effect on ER performance of composite nanoplates,
2.1.4 Polyaniline/polymer composites
For ER application, the conductivity of PAn has to be adjusted to desired level to prevent electrical short upon application of the
2.1.5 Polyaniline/poly(ionic liquid) composites
Organic polymer-based materials have been most frequently studied as ER materials compared to the inorganic ones due to their low density, soft texture, and relatively high ER activity. Among various organic polymer-based polymers, polyelectrolytes are more extensively applied in ER fluids due to their low cost, facile fabrication, and high ER response in the presence of promoter, such as a small amount of water or moisture. The small amount of water required for ER activation leads the traditional polyelectrolytes to face the electrical and thermal problems. To overcome these drawbacks, a novel anhydrous polyelectrolyte-based ER system based on hydrophobic poly(ionic liquid)s (PILs) has been developed recently [104].
PILs are a type of functional polyelectrolyte obtained by polymerization of ionic liquids, a typical organic molten salt bearing hydrophobic counterions at room temperature. PILs can exhibit strong ER activity and high dielectric polarizability without affinity to water due to comprising of high-density cation/anion counterions endowing polarized easily upon application of the
At higher
The influence of oxidation state of PAn on the ER response of PIL/PAn composite composed of P[VBTMA][PF6] as a matrix and different forms of semiconducting PAn as a filler, prepared by ion-exchange and subsequently treated by ammonia or hydrazine to obtain different forms of PAn filler, such as emeraldine salt, emeraldine base, and leucoemeraldine was studied by Zheng et al. Thus, the researchers could adjust the difference in polarization rate between filler and matrix and reported that when the closer their polarization rates were, the more stable the flow curve was in a broad shear rate region, and enhanced ER response was achieved only for the ammonia-treated P[VBTMA][PF6]/PAn particles at room temperature. On the other hand, the flow curves of the hydrazine-treated P[VBTMA][PF6]/PAn displayed more stable flow curve with increasing temperature [109]. In another study, ionic liquid crystal PAn prepared
2.2 ER characteristics of polyaniline-based materials
Within the realm of ER fluids, the ER characteristics have been extensively examined by rheological measurements including steady shear, on/off switch test at constant shear rate, and oscillatory test under

Figure 2.
Representative shear stress versus shear rate curves of an ER fluid that fits the Newtonian model under off-field, the Bingham or CCJ model under on-field, and corresponding schematic microstructure under shear flow (a) and representative dielectric spectrum (b) of an ER fluid.
The viscoelastic properties and phase transition of ER fluids can be examined by dynamic oscillation test in which a sinusoidal strain or stress is applied, and the ER fluid is sheared back and forth at a given strain amplitude and frequency [5]. In order to do so, a linear viscoelastic region (LVE), where stress and strain are proportional, is initially determined by stress or strain amplitude sweep test at a fixed frequency value. Within the low-strain region, elastic (G′) and viscous (G″) modulus are independent of the applied strain or stress and show a constant plateau. When the applied strain or stress is inadequate and leads to structural breakdown of the field-induced structures, the important microstructural properties are being measured. Out of the LVE, nonlinearities arise and measurements can no longer be easily correlated with microstructural properties. Within the predetermined LVE for the given ER fluid, the angular frequency sweep test is performed to analyze the time-dependent behavior of the ER fluid in the nondestructive deformation range and to determine the behavior and inner structure, and the long-term stability of the ER fluid [113]. In the absence of the
Furthermore, the microstructural changes in the ER fluid can be directly determined by optical microscopy under
Dielectric spectra of ER fluids, the frequency-dependent dielectric constant (ε′) and dielectric loss factor (ε″) curves (Figure 2b), provide important information about the polarization mechanism, polarizability, and polarization relaxation time (λ) of ER fluids on analyzing of electrical polarization properties and interpreting the flow behavior under the
3. Polyaniline-based MR fluids
MR fluids whose rheological properties tuned reversibly and rapidly (in a fraction of millisecond) from a liquid to a nearly solid state under the presence of the
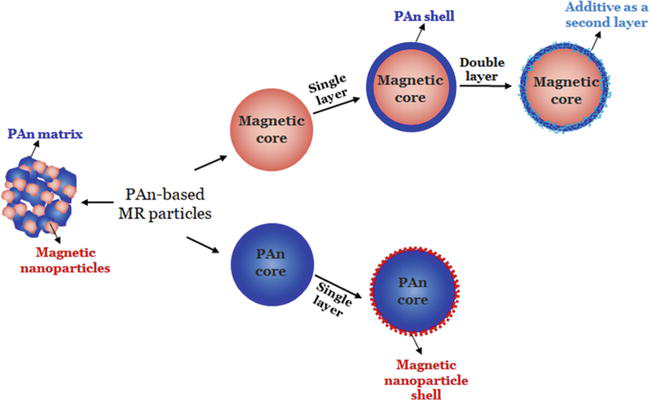
Figure 3.
Synthetic approaches for the preparation of PAn-based MR particles.
Material | Magnetic particle (its Ms) | Additive | Morphology | Synthesis method | Density (g/cm3) | Ms | MR fluid concentration | Optimum H | Yield stress at optimum H | Index α | Dual response | Ref |
---|---|---|---|---|---|---|---|---|---|---|---|---|
PAn@Fe3O4 | Fe3O4 (70 emu/g) | — | Nano-sized Fe3O4-coated PAn tubes, approximately 148 nm in diameter and several μm in length | Self-assembly method | — | 39.4 emu/g | 2 vol% in SO | 342 kA/m | Approximately 60 Pa | 1.5 | — | [117] |
PAn/nano-sized Fe3O4 composites | Fe3O4 (60 emu/g) | — | Nano-sized Fe3O4 particles embedded into the PAn matrix | Chemical reaction method | — | 18 emu/g | 30 vol% in SO | 343 kA/m | — | — | — | [118] |
MWCNT/PAn/CI | CI | — | Micron-sized spherical, MWCNT wrapped PAn coated CI particles with rough surface | Two-step process: i. Conventional dispersion polymerization, ii. Solvent casting method | 6.70 | — | — | 343 kA/m | 9.497 kPa | 1.79 | — | [114] |
PAn/CI | CI | 0.5 wt% of nano-silica | Micron-sized spherical core–shell composite particles with a CI core and PAn shell | Coating of CI surface using a PAn colloidal dispersion prepared by emulsion polymerization | — | — | 80 wt% in SO | 307 mT | Approximately 8 kPa | 1.5-2 | — | [115] |
MWCNT/PAn/CI | CI (188 Am2/kg) | — | MWCNT nest on the PAn-coated CI core/shell spherical particles | Dispersion polymerization followed by facile solvent casting | 6.70 | 161 Am2/kg | 20 vol% in SO | 343 kA/m | 10 kPa | 2 at low fields and 1.5 at high fields | — | [116] |
Cl/PAn/Fe | Cl microparticles and Fe nanoparticles | PAn/Fe | PAn/Fe composite nanofibers approximately 80–150 nm in diameter with rough surface used as additive for microspherical Cl | Two-step process for additive: i. Rapid mixing, oxidative polymerization, ii. Reduction of the polymerization by-products FeCl2/FeCl3 by NaBH4 solution | — | (23.1 emu/g for additive PAn/Fe) | 70 wt% Cl and 0.1 wt% PAn/Fe nanofibers | 343 kA/m | 104 Pa | 2 at low fields and 1.5 at high fields | — | [119] |
Cl/PAn | Cl (204 emu/g) | — | Cl core and PAn shell with polydisperse size distribution and rough surface | Dispersion polymerization | 7.05 | 185 emu/g | 20 vol% in SO | 342 kA/m | 12.5 kPa | — | [120] | |
CI/PAn | Cl (195 emu/g) | — | Microspherical CI/PAn particles with rough surfaces and some aggregated particles | 4.21 | 136 emu/g | 10 vol% in SO | 343 kA/m | 401 Pa (Herschel-Bulkley model) | 2.0 | — | [121] | |
PAn/ ZnFe2O4 | ZnFe2O4 (91 emu/g) | — | Raspberry-like core−shell composite consisting of PAn core and ZnFe2O4 shell | Pickering emulsion polymerization | 5.4 | 73.7 emu/g | 5 vol% in SO | 171 kA/m | Approximately 140 Pa | 1.0 | Dual | [122] |
ZnFe2O4/PAn | ZnFe2O4 (73.67 emu/g) | — | ZnFe2O4 core with approximately 340 nm size coated with approximately 30 nm PAn shell | 2.4 | 43.93 emu/g | 5 vol% in SO | 274 kA/m | 48.66 Pa | 1.0 | Dual | [123] | |
ZnFe2O4/Poly(N-methyl aniline) | ZnFe2O4 (73.67 emu/g) | — | Microspherical ZnFe2O4 core coated with poly(N-methyl aniline), approximately 428 nm particle size with rough surface | 2.1 | 39.93 emu/g | 5 vol% in SO | 171 kA/m | 47.75 Pa | — | Dual | [124] | |
ZnFe2O4/Poly(diphenylamine) | ZnFe2O4 (83.4 emu/g) | — | Core/shell particles with diameter of approximately 300 nm and rough surface | Radical polymerization of polymer through surface-modified ZnFe2O4 by a grafting agent | 2.7 | 40.8 emu/g | 5 vol% in SO | 205 kA/m | Approximately 102 Pa | 0.5 | Dual | [125] |
Poly(N-methylaniline)/Fe3O4 | Fe3O4 nanoparticles | — | Poly(N-methylaniline) microsphere coated with nano-sized Fe3O4 shell | Chemical co-precipitation method of Fe3O4 in the presence of poly(N-methyl aniline) | 1.97 | 32.1 emu/g | 10 vol% in SO | 257 kA/m | 177.4 Pa (Herschel-Bulkley model) | 1.0 | Dual | [126] |
MnFe2O4/PAn | MnFe2O4 (49.97 emu/g) | — | MnFe2O4/PAn particles with approximately 450 nm size, and approximately 25 nm coating thickness | 1.89 | 27.46 emu/g | 5 vol% in SO | 171 kA/m | Approximately 20 Pa | 1.0 | Dual | [127] |
Table 1.
3.1 Development and diversification of polyaniline-based MR fluids
Various soft-magnetic particles such as Fe3O4, carbonyl iron (CI), CoNi, zinc-ferrite (ZnFe2O4), and MnFe2O4 have been used as a dispersed phase for MR fluids. Their organic/inorganic composites have attracted considerable interest in terms of increasing dispersion stability of fluid, protecting magnetic particles against corrosion, and improving reversible behavior in field on/off states while maintaining intrinsic MR properties of soft-magnetic particles. However, fabricating hybrid structures have their pros and cons. Hybrid structures show slightly inferior magnetic properties compared to bare soft-magnetic particles as the aforementioned improvements are gained.
PAn having fibrous structure was coated with Fe3O4 by an
CI particles obtained from the decomposition of iron pentacarbonyl are most used for MR fluids due to their large saturation magnetization, small coercivity magnetization, and appropriate size. However, CI-based MR fluids generally have serious sedimentation problems due to the large density mismatch between the CI particles and the carrier fluid. One of the strategies to reduce the density or prevent particle aggregation is polymer coating on the particle surface. PAn coating has been introduced to reduce the density or prevent CI particle aggregation. Core-shell structured CI-PAn composite particles, prepared by dispersing CI particles in pre-synthesized PAn colloidal dispersion in chloroform, were reported to have similar magnetic properties with CI particles whereas enhanced dispersion stability, decreased field off viscosity and increased MR effect were observed compared to bare CI MR fluid [115]. To increase interfacial interaction and affinity between magnetic particles and PAn, Cl surface can be modified by suitable grafting agents. In a study, dopamine-attached CI particles were coated by PAn by dispersion polymerization process and the formed homogenous rough surface and low density of the core/shell PAn/CI particles resulted in improved dispersion stability [120]. For the same purposes, in another study,
Magnetic ZnFe2O4 particles coated with conducting PAn particles with raspberry-like core/shell morphology synthesized by Pickering emulsion polymerization were reported to exhibit dual ER/MR active material [122]. In another study, rough surfaces were observed when ZnFe2O4 was coated with dedoped-PAn with dual-field responsive properties [123]. Also, PAn derivatives poly(N-methyl aniline) [124] and poly(diphenylamine) [125] were also reported to be as a shell layer on magnetic ZnFe2O4 particles without any requirement of an additional de-doping process. PAn-coated spherical MnFe2O4 nanoparticles were reported with dual characteristics of ER/MR fluids [127].
3.2 MR characteristics of polyaniline-based materials
MR fluids often show a Newtonian fluid behavior, in which shear stress increases linearly with shear rate without the
4. Conclusions
This chapter presents an overview of the status and recent studies in PAn-based materials used in electro/magneto-responsive smart fluids. Electro/magneto-responsive smart fluids have tremendous applications such as shock absorbers, robotics, clutches, valves, dampers, and microfluidics due to their possessing an ability to change their rheological properties reversibly and promptly under externally applied electric or magnetic fields in a controlled manner. The electro-responsive smart fluid, namely, ER fluids mainly consists of polarizable particles dispersed in nonconductive liquid dispersant and furthermore, various polar additives may be inserted to increase polarizability or dispersion stability of the ER fluid. But, instead of incorporating additives due to their drawbacks such as leading electrical breakdown and device corrosion, inherently anhydrous polarizable particles are desired to be used as an ER active material. Thus, inherently polarizable PAn has been one of the most promising and extensively studied conducting polymers in the research of ER materials for many years due to its tunable conductivity, ease of synthesis, adequate chemical and thermal stability, noncorrosiveness, and less friction than pure inorganic compounds. The morphology, size, shape, surface characteristics, dedoping level, and incorporation of other compounds with PAn have a significant influence on the ER properties. In this chapter, PAn-based ER fluids with various compositions are categorized in terms of constituent components and discussed in detail. Various inorganic compounds, insulating polymers, PILs, and carbon-based nanomaterials have been hybridized with PAn and PAn derivatives to improve the ER performance and the dispersion stability.
The magnetic field analog of ER fluids, MR fluids are basically made up of soft magnetizable dispersed particles, carrier fluid, and additives. The researchers have focused on enhancing the dispersion stability of soft-magnetizable dispersed particles in MR fluid, preventing particle corrosion, and improving the overall MR effect by reduction in density mismatch between carrier fluid and dispersed particles, the addition of various additives and particle coating with inorganic, organic or polymeric components. Even though PAn has no soft magnetic properties, PAn and its derivatives have also been used for enhancing the dispersion stability of MR fluids and obtaining dual ER/MR response. For that reason, there have been fewer studies on the use of PAn in MR fluids compared to its use in the ER fluids. The literature studies have indicated that while introducing PAn in MR materials enhanced the sedimentation stability, the magnetic saturation of the resulting structure was decreased. Therefore, there are still major opportunities for the development of PAn with low magnetic masking property in the future research.
According to the recent studies reported so far, it has been concluded that owing high surface area, good wettability, rough surface compared to smooth surface, hierarchical anisotropic structures compared to isotropic ones, suitable conductivity, and reduced density mismatch with carrier fluid led to enhanced ER/MR performances of PAn-based stimuli responsive fluids.
References
- 1.
Hao T. Electrorheological fluids. Advanced Materials. 2001; 13 (24):1847-1857 - 2.
de Vicente J, Klingenberg DJ, Hidalgo-Alvarez R. Magnetorheological fluids: A review. Soft Matter. 2011; 7 (8):3701-3710 - 3.
Winslow WM. Induced fibration of suspensions. Journal of Applied Physics. 1949; 20 (12):1137-1140 - 4.
Hao T. Electrorheological suspensions. Advances in Colloid and Interface Science. 2002; 97 (1):1-35 - 5.
Dong YZ, Kim HM, Choi HJ. Conducting polymer-based electro-responsive smart suspensions. Chemical Papers. 2021; 75 (10):5009-5034 - 6.
Kumar JS, Paul PS, Raghunathan G, Alex DG. A review of challenges and solutions in the preparation and use of magnetorheological fluids. International Journal of Mechanical and Materials Engineering. 2019; 14 (1):13 - 7.
Rabbani Y, Hajinajaf N, Shariaty-Niassar M. The effect of microparticles/nanoparticles surface modification on the magnetorheological fluid properties: A review. Journal of Intelligent Material Systems and Structures. 2023; 0 (0):1045389X221147667 - 8.
Kuznetsov NM, Kovaleva VV, Belousov SI, Chvalun SN. Electrorheological fluids: from historical retrospective to recent trends. Materials Today Chemistry. 2022; 26 :101066 - 9.
Stenicka M, Pavlinek V, Saha P, Blinova NV, Stejskal J, Quadrat O. The effect of compatibility of suspension particles with the oil medium on electrorheological efficiency. Journal of Intelligent Material Systems and Structures. 2012; 23 (9):1055-1059 - 10.
Stěnička M, Pavlínek V, Sáha P, Blinova NV, Stejskal J, Quadrat O. Effect of hydrophilicity of polyaniline particles on their electrorheology: Steady flow and dynamic behaviour. Journal of Colloid and Interface Science. 2010; 346 (1):236-240 - 11.
Xie H-Q, Guan J-G, Guo J-S. Three ways to improve electrorheological properties of polyaniline-based suspensions. Journal of Applied Polymer Science. 1997; 64 :1641-1647 - 12.
Sebastian J, Samuel JM. Recent advances in the applications of substituted polyanilines and their blends and composites. Polymer Bulletin. 2020; 77 (12):6641-6669 - 13.
Fang FF, Lee BM, Choi HJ. Electrorheologically intelligent polyaniline and its composites. Macromolecular Research. 2010; 18 (2):99-112 - 14.
Kuramoto N, Takahashi Y, Nagai K, Koyama K. Electrorheological properties of poly(o-anisidine) and poly(o-anisidine)-coated silica suspensions. Reactive and Functional Polymers. 1996; 30 (1):367-373 - 15.
Liu J, Wen X, Liu Z, Tan Y, Yang S, Zhang P. Electrorheological performances of poly(o-toluidine) and p-toluenesulfonic acid doped poly(o-toluidine) suspensions. Colloid and Polymer Science. 2015; 293 (5):1391-1400 - 16.
Gercek B, Yavuz M, Yilmaz H, Sari B, Unal HI. Comparison of electrorheological properties of some polyaniline derivatives. Colloids and Surfaces A: Physicochemical and Engineering Aspects. 2007; 299 (1):124-132 - 17.
Kim JW, Jang WH, Choi HJ, Joo J. Synthesis and electrorheological characteristics of polyaniline derivatives with different substituents. Synthetic Metals. 2001; 119 (1):173-174 - 18.
Kim MH, Bae DH, Choi HJ, Seo Y. Synthesis of semiconducting poly(diphenylamine) particles and analysis of their electrorheological properties. Polymer. 2017; 119 :40-49 - 19.
Woo DJ, Suh MH, Shin ES, Lee CW, Lee SH. Electrorheological behavior of suspensions of a substituted polyaniline with long alkyl pendants. J Colloid Interface Sci. 2005; 288 (1):71-74 - 20.
Han WJ, Choi HJ. Synthesis of conducting polymeric nanoparticles in the presence of a polymerizable surfactant and their electrorheological response. Colloid and Polymer Science. 2019; 297 (5):781-784 - 21.
Choi HJ, Kim JW, To K. Electrorheological characteristics of semiconducting poly(aniline-co-o-ethoxyaniline) suspension. Polymer. 1999; 40 (8):2163-2166 - 22.
Trlica J, Sáha P, Quadrat O, Stejskal J. Electrical and electrorheological behavior of poly(aniline-co-1,4-phenylenediamine) suspensions. European Polymer Journal. 2000; 36 (11):2313-2319 - 23.
Cho MS, Kim TW, Choi HJ, Jhon MS. N-substituted copolyaniline for electrorheological material. Journal of Materials Science Letters. 1997; 16 :672-673 - 24.
Cho CH, Choi HJ, Kim JW, Jhon MS. Synthesis and electrorheology of aniline/pyrrole copolymer. Journal of Materials Science. 2004; 39 (5):1883-1885 - 25.
Mrlik M, Sedlacik M, Pavlinek V, Bober P, Trchová M, Stejskal J, et al. Electrorheology of aniline oligomers. Colloid and Polymer Science. 2013; 291 (9):2079-2086 - 26.
Sung BH, Choi US, Jang HG, Park YS. Novel approach to enhance the dispersion stability of ER fluids based on hollow polyaniline sphere particle. Colloids and Surfaces A: Physicochemical and Engineering Aspects. 2006; 274 (1):37-42 - 27.
Yin J, Zhao X, Xia X, Xiang L, Qiao Y. Electrorheological fluids based on nano-fibrous polyaniline. Polymer. 2008; 49 (20):4413-4419 - 28.
Fang FF, Dong Y-Z, Choi HJ. Effect of oxidants on morphology of interfacial polymerized polyaniline nanofibers and their electrorheological response. Polymer. 2018; 158 :176-182 - 29.
Yin J, Xia X, Xiang L, Zhao X. Conductivity and polarization of carbonaceous nanotubes derived from polyaniline nanotubes and their electrorheology when dispersed in silicone oil. Carbon. 2010; 48 (10):2958-2967 - 30.
Lee BM, Kim JE, Fang FF, Choi HJ, Feller J-F. Rectangular-shaped polyaniline tubes covered with nanorods and their electrorheology. Macromolecular Chemistry and Physics. 2011; 212 (21):2300-2307 - 31.
Kim MJ, Liu YD, Choi HJ. Urchin-like polyaniline microspheres fabricated from self-assembly of polyaniline nanowires and their electro-responsive characteristics. Chemical Engineering Journal. 2014; 235 :186-190 - 32.
Wen Q, He K, Wang C, Wang B, Yu S, Hao C, et al. Clip-like polyaniline nanofibers synthesized by an insitu chemical oxidative polymerization and its strong electrorheological behavior. Synthetic Metals. 2018; 239 :1-12 - 33.
Yin J, Xia X, Xiang L, Qiao Y, Zhao X. The electrorheological effect of polyaniline nanofiber, nanoparticle and microparticle suspensions. Smart Materials and Structures. 2009; 18 (9):095007 - 34.
Lin DD, Zhang ZJ, Zhao BY, Chen LS, Hu K. Rapid synthesis of porous polyaniline and its application in electrorheological fluid. Smart Materials and Structures. 2006; 15 (6):1641 - 35.
Choi HJ, Cho MS, To K. Electrorheological and dielectric characteristics of semiconductive polyaniline-silicone oil suspensions. Physica A: Statistical Mechanics and its Applications. 1998; 254 (1):272-279 - 36.
Liu YD, Choi HJ. Electrorheological response of polyaniline and its hybrids. Chemical Papers. 2013; 67 (8):849-859 - 37.
Ji L, Zhang J. Synthesis, characterization and electrorheological properties of polyaniline/titanate core-shell composite. Journal of Macromolecular Science, Part A. 2009; 46 (7):688-693 - 38.
Cheng Q, Pavlinek V, He Y, Li C, Saha P. Electrorheological characteristics of polyaniline/titanate composite nanotube suspensions. Colloid and Polymer Science. 2009; 287 (4):435-441 - 39.
Yin J, Xia X, Wang X, Zhao X. The electrorheological effect and dielectric properties of suspensions containing polyaniline@titania nanocable-like particles. Soft Matter. 2011; 7 (22):10978-10986 - 40.
Wang B, Liu C, Yin Y, Yu S, Chen K, Liu P, et al. Double template assisting synthesized core–shell structured titania/polyaniline nanocomposite and its smart electrorheological response. Composites Science and Technology. 2013; 86 :89-100 - 41.
Lee IS, Lee JY, Sung JH, Choi HJ. Synthesis and electrorheological characteristics of polyaniline-titanium dioxide hybrid suspension. Synthetic Metals. 2005; 152 (1):173-176 - 42.
Tang J, Wen X, Liu Z, Wang J, Zhang P. Synthesis and electrorheological performances of 2D PANI/TiO2 nanosheets. Colloids and Surfaces A: Physicochemical and Engineering Aspects. 2018; 552 :24-31 - 43.
Lee S, Noh J, Jekal S, Kim J, Oh W-C, Sim H-S, et al. Hollow TiO2 nanoparticles capped with polarizability-tunable conducting polymers for improved electrorheological activity. Nanomaterials. 2022; 12 (19):3521 - 44.
Wang B, Liu C, Yin Y, Tian X, Yu S, Chen K, et al. The electrorheological properties of polyaniline nanofiber/kaolinite hybrid nanocomposite. Journal of Applied Polymer Science. 2013; 130 (2):1104-1113 - 45.
Lengálová A, Vr P, Sáha P, Stejskal J, Kitano T, Quadrat O. The effect of dielectric properties on the electrorheology of suspensions of silica particles coated with polyaniline. Physica A: Statistical Mechanics and its Applications. 2003; 321 (3):411-424 - 46.
Liu YD, Zhang WL, Choi HJ. Pickering emulsion polymerization of core–shell-structured polyaniline@SiO2 nanoparticles and their electrorheological response. Colloid and Polymer Science. 2012; 290 (9):855-860 - 47.
Jang HS, Kwon SH, Lee JH, Choi HJ. Facile fabrication of core-shell typed silica/poly(diphenylamine) composite microparticles and their electro-response. Polymer. 2019; 182 :121851 - 48.
Zhang WL, Choi HJ. Fabrication of semiconducting polyaniline-wrapped halloysite nanotube composite and its electrorheology. Colloid and Polymer Science. 2012; 290 (17):1743-1748 - 49.
Marins JA, Giulieri F, Soares BG, Bossis G. Hybrid polyaniline-coated sepiolite nanofibers for electrorheological fluid applications. Synthetic Metals. 2013; 185-186 :9-16 - 50.
Jang DS, Choi HJ. Conducting polyaniline-wrapped sepiolite composite and its stimuli-response under applied electric fields. Colloids and Surfaces A: Physicochemical and Engineering Aspects. 2015; 469 :20-28 - 51.
Tian X, He K, Wang B, Yu S, Hao C, Chen K, et al. Flower-like Fe2O3/polyaniline core/shell nanocomposite and its electroheological properties. Colloids and Surfaces A: Physicochemical and Engineering Aspects. 2016; 498 :185-193 - 52.
Zhu X, Zhao Q, Zhang T, Pang X. Electrorheological response of novel polyaniline-Fe2O3 nanocomposite particles. Polymer-Plastics Technology and Materials. 2019; 58 (5):573-577 - 53.
Dong YZ, Choi HJ. Electrorheological characteristics of poly(diphenylamine)/magnetite composite-based suspension. Materials. 2019; 12 (18):2911 - 54.
Lu Q, Lee JH, Lee JH, Choi HJ. Magnetite/poly(ortho-anisidine) composite particles and their electrorheological response. Materials. 2021; 14 (11):2900 - 55.
Chae HS, Zhang WL, Piao SH, Choi HJ. Synthesized palygorskite/polyaniline nanocomposite particles by oxidative polymerization and their electrorheology. Applied Clay Science. 2015; 107 :165-172 - 56.
Fei Fang F, Hye Kim J, Jin Choi H, Seo Y. Organic/inorganic hybrid of polyaniline/BaTiO3 composites and their electrorheological and dielectric characteristics. Journal of Applied Polymer Science. 2007; 105 (4):1853-1860 - 57.
Yan H, Liao ZJ, Zhu X, Wang XM, Chen Y, Zhang B, et al. Synthesis and electrorheological properties of polyaniline-coated barium titanate composite particles. Journal of Applied Polymer Science. 2008; 107 (3):1960-1966 - 58.
Linzhi LI, Shujuan GAO. Polyaniline (PANI) and BaTiO3 composite nanotube with high suspension performance in electrorheological fluid. Materials Today Communications. 2020; 24 :100993 - 59.
Wang B, Yin Y, Liu C, Yu S, Chen K. Synthesis and characterization of clay/polyaniline nanofiber hybrids. Journal of Applied Polymer Science. 2013; 128 (2):1304-1312 - 60.
Kim JW, Kim SG, Choi HJ, Jhon MS. Synthesis and electrorheological properties of polyaniline-Na+-montmorillonite suspensions. Macromolecular Rapid Communications. 1999; 20 (8):450-452 - 61.
Lu J, Zhao X. Electrorheological properties of a polyaniline–montmorillonite clay nanocomposite suspension. Journal of Materials Chemistry. 2002; 12 (9):2603-2605 - 62.
Song DH, Lee HM, Lee K-H, Choi HJ. Intercalated conducting polyaniline–clay nanocomposites and their electrical characteristics. Journal of Physics and Chemistry of Solids. 2008; 69 (5):1383-1385 - 63.
Lim YT, Park JH, Park OO. Improved electrorheological effect in polyaniline nanocomposite suspensions. Journal of Colloid and Interface Science. 2002; 245 (1):198-203 - 64.
Fang FF, Liu YD, Choi HJ. Synthesis and electrorheological characteristics of polyaniline/organoclay nanoparticles via Pickering emulsion polymerization. Smart Materials and Structures. 2010; 19 (12):124002 - 65.
Sung JH, Choi HJ. Effect of pH on physical characteristics of conducting poly(o-ethoxyaniline) nanocomposites. Journal of Macromolecular Science, Part B. 2005; 44 (3):365-375 - 66.
Jun CS, Sim B, Choi HJ. Fabrication of electric-stimuli responsive polyaniline/laponite composite and its viscoelastic and dielectric characteristics. Colloids and Surfaces A: Physicochemical and Engineering Aspects. 2015; 482 :670-677 - 67.
Cho MS, Choi HJ, Kim KY, Ahn WS. Synthesis and characterization of polyaniline/mesoporous SBA-15 nanocomposite. Macromolecular Rapid Communications. 2002; 23 (12):713-716 - 68.
Cho MS, Choi HJ, Ahn W-S. Enhanced electrorheology of conducting polyaniline confined in MCM-41 channels. Langmuir. 2004; 20 (1):202-207 - 69.
Fang FF, Choi HJ, Ahn WS. Electroactive response of mesoporous silica and its nanocomposites with conducting polymers. Composites Science and Technology. 2009; 69 (13):2088-2092 - 70.
Noh J, Yoon C-M, Jang J. Enhanced electrorheological activity of polyaniline coated mesoporous silica with high aspect ratio. Journal of Colloid and Interface Science. 2016; 470 :237-244 - 71.
Wei C, Zhu Y, Yang X, Li C. One-pot synthesis of polyaniline-doped in mesoporous TiO2 and its electrorheological behavior. Materials Science and Engineering: B. 2007; 137 (1):213-216 - 72.
Tian X, He K, Wang C, Wen Q, Wang B, Yu S, et al. Preparation and electrorheological behavior of anisotropic titanium oxide/polyaniline core/shell nanocomposite. Composites Science and Technology. 2016; 137 :118-129 - 73.
Han WJ, Piao SH, Choi HJ. Synthesis and electrorheological characteristics of polyaniline@attapulgite nanoparticles via Pickering emulsion polymerization. Materials Letters. 2017; 204 :42-44 - 74.
Chattopadhyay A, Rani P, Srivastava R, Dhar P. Electro-elastoviscous response of polyaniline functionalized nano-porous zeolite based colloidal dispersions. Journal of Colloid and Interface Science. 2018; 519 :242-254 - 75.
Wen Q, Ma L, Wang C, Wang B, Han R, Hao C, et al. Preparation of core–shell structured metal–organic framework@PANI nanocomposite and its electrorheological properties. RSC Advances. 2019; 9 (25):14520-14530 - 76.
Zhang WL, Jiang D, Wang X, Hao BN, Liu YD, Liu J. Growth of polyaniline nanoneedles on MoS2 nanosheets, tunable electroresponse, and electromagnetic wave attenuation analysis. The Journal of Physical Chemistry C. 2017; 121 (9):4989-4998 - 77.
Li C, Chen Y, Wang L, Wang Z, Lin Y, Xiong K, et al. Electrorheological response behavior of PANI@MoS2 core–shell nanocomposites. Advanced Engineering Materials. 2023; 25 :2300029 - 78.
Stejskal J, Mrlík M, Plachý T, Trchová M, Kovářová J, Li Y. Molybdenum and tungsten disulfides surface-modified with a conducting polymer, polyaniline, for application in electrorheology. Reactive and Functional Polymers. 2017; 120 :30-37 - 79.
Erol O, Karakisla M, Unal HI, Sacak M. Electrorheological properties of polyaniline/K-feldspar conducting composite. Journal of Composite Materials. 2012; 46 (11):1295-1304 - 80.
Yavuz M, Gok A, Sen S, Unal HI. Electrorheological properties of polyaniline/red mud composite. International Journal of Polymer Analysis and Characterization. 2008; 13 (1):9-24 - 81.
Lengálová A, Pavlı́nek Vr, Sáha P, Stejskal J, Quadrat O. Electrorheology of polyaniline-coated inorganic particles in silicone oil. Journal of Colloid and Interface Science. 2003; 258 (1):174-178 - 82.
Munteanu L, Munteanu A, Sedlacik M, Kutalkova E, Kohl M, Kalendova A. Zinc ferrite/polyaniline composite particles: Pigment applicable as electro-active paint. J Ind Eng Chem. 2022; 115 :440-448 - 83.
Zhang WL, Liu J, Choi HJ. Graphene and graphene oxide composites and their electrorheological applications. Journal of Nanomaterials. 2015; 2015 :574637 - 84.
Lu Q, Jang HS, Han WJ, Lee JH, Choi HJ. Stimuli-responsive graphene oxide-polymer nanocomposites. Macromolecular Research. 2019; 27 (11):1061-1070 - 85.
Park SJ, Park SY, Cho MS, Choi HJ, Jhon MS. Synthesis and electrorhelogy of multi-walled carbon nanotube/polyaniline nanoparticles. Synthetic Metals. 2005; 152 (1):337-340 - 86.
Sim B, Zhang WL, Choi HJ. Graphene oxide/poly(2-methylaniline) composite particle suspension and its electro-response. Materials Chemistry and Physics. 2015; 153 :443-449 - 87.
Zhang K, Li H, Dong YZ, Zhang H, Zhao W, Zhao S, et al. Jellyfish-shaped p-phenylenediamine functionalized graphene oxide-g-polyaniline fibers and their electrorheology. Polymer. 2019; 168 :29-35 - 88.
Gao CY, Kim MH, Jin H-J, Choi HJ. Synthesis and electrorheological response of graphene oxide/polydiphenylamine microsheet composite particles. Polymers. 2020; 12 (9):1984 - 89.
Yin J, Wang X, Chang R, Zhao X. Polyaniline decorated graphene sheet suspension with enhanced electrorheology. Soft Matter. 2012; 8 (2):294-297 - 90.
Yuan J, Wang Y, Xiang L, Zhao X, Yin J. Understanding the enhanced electrorheological effect of reduced graphene oxide-supported polyaniline dielectric nanoplates by a comparative study with graphene oxide as the support core. IET Nanodielectrics. 2021; 4 (3):143-154 - 91.
Sedlacik M, Almajdalawi S, Mrlik M, Pavlinek V, Saha P, Stejskal J. Viscoelastic properties of electrorheological suspensions of core-shell (carbon/polyaniline) particles in silicone oil. Journal of Physics: Conference Series. 2013; 412 (1):012006 - 92.
Yin J, Shui Y, Chang R, Zhao X. Graphene-supported carbonaceous dielectric sheets and their electrorheology. Carbon. 2012; 50 (14):5247-5255 - 93.
Sedlacik M, Pavlinek V, Mrlik M, Morávková Z, Hajná M, Trchová M, et al. Electrorheology of polyaniline, carbonized polyaniline, and their core–shell composites. Materials Letters. 2013; 101 :90-92 - 94.
Abu Hassan Shaari H, Ramli MM, Mohtar MN, Abdul Rahman N, Ahmad A. Synthesis and conductivity studies of poly(methyl methacrylate) (PMMA) by co-polymerization and blending with polyaniline (PANi). Polymers. 2021; 13 (12):1939 - 95.
Kim MH, Choi HJ. Core–shell structured semiconducting poly(diphenylamine)-coated polystyrene microspheres and their electrorheology. Polymer. 2017; 131 :120-131 - 96.
Sung SK, Hyoung JC. Poly(2,5-dimethoxyaniline) coated polystyrene microspheres and their electrorheological characteristics. Colloids and Surfaces A: Physicochemical and Engineering Aspects. 2020; 605 :125375 - 97.
Park SY, Cho MS, Kim CA, Choi HJ, Jhon MS. Polyaniline microsphere encapsulated by poly(methyl methacrylate) and investigation of its electrorheological properties. Colloid and Polymer Science. 2003; 282 (2):198-202 - 98.
Liu YD, Fang FF, Choi HJ. Core−shell structured semiconducting PMMA/polyaniline snowman-like anisotropic microparticles and their electrorheology. Langmuir. 2010; 26 (15):12849-12854 - 99.
Lee IS, Cho MS, Choi HJ. Preparation of polyaniline coated poly(methyl methacrylate) microsphere by graft polymerization and its electrorheology. Polymer. 2005; 46 (4):1317-1321 - 100.
Jun J-B, Kim J-W, Suh K-D. Monodisperse micron-sized polyaniline composite particles for electrorheological fluid material. Macromolecular Chemistry and Physics. 2002; 203 (7):1011-1017 - 101.
Cho MS, Cho YH, Choi HJ, Jhon MS. Synthesis and electrorheological characteristics of polyaniline-coated poly(methyl methacrylate) microsphere: Size effect. Langmuir. 2003; 19 (14):5875-5881 - 102.
Moon IJ, Choi HJ. Core-shell-structured copolyaniline-coated polymeric nanoparticle suspension and its viscoelastic response under various electric fields. Materials. 2015; 8 (8):4932-4942 - 103.
Jun CS, Kwon SH, Choi HJ, Seo Y. Polymeric nanoparticle-coated Pickering emulsion-synthesized conducting polyaniline hybrid particles and their electrorheological study. ACS Applied Materials & Interfaces. 2017; 9 (51):44811-44819 - 104.
Dong Y, Yin J, Zhao X. Microwave-synthesized poly(ionic liquid) particles: A new material with high electrorheological activity. Journal of Materials Chemistry A. 2014; 2 (25):9812-9819 - 105.
Dong Y, Liu Y, Wang B, Xiang L, Zhao X, Yin J. Influence of counterion type on dielectric and electrorheological responses of poly(ionic liquid)s. Polymer. 2017; 132 :273-285 - 106.
Liu Y, Zhao J, He F, Zheng C, Zhao X, Yin J. Influence of alkyl spacer length on ion transport, polarization and electro-responsive electrorheological effect of self-crosslinked poly(ionic liquid)s. Polymer. 2019; 171 :161-172 - 107.
Zheng C, Dong Y, Liu Y, Zhao X, Yin J. Enhanced stimuli-responsive electrorheological property of poly(ionic liquid)s-capsulated polyaniline particles. Polymers. 2017; 9 (9):385 - 108.
Zheng C, Liu Y, Dong Y, He F, Zhao X, Yin J. Low-temperature interfacial polymerization and enhanced electro-responsive characteristic of poly(ionic liquid)s@polyaniline core-shell microspheres. Macromolecular Rapid Communications. 2019; 40 (17):1800351 - 109.
Zheng C, Lei Q, Zhao J, Zhao X, Yin J. The effect of dielectric polarization rate difference of filler and matrix on the electrorheological responses of poly(ionic liquid)/polyaniline composite particles. Polymers. 2020; 12 (3):703 - 110.
Li X, Yan G, Wang J, Kong W, Chang X, Zhuang Y, et al. Effect of a temperature threshold on the electrorheological performance of ionic liquid crystal polyanilines. Journal of Molecular Liquids. 2021; 326 :115299 - 111.
Meng F-B, Zhou N-Y, Du C, Gao H-M, He X-Z. Synthesis, characterization and electrorheological effect of sulfosalt-type liquid-crystalline ionomers containing polyaniline units. Journal of Applied Polymer Science. 2013; 130 (5):3395-3403 - 112.
Seo YP, Choi HJ, Lee JR, Seo Y. Modeling and analysis of an electrorheological flow behavior containing semiconducting graphene oxide/polyaniline composite particles. Colloids and Surfaces A: Physicochemical and Engineering Aspects. 2014; 457 :363-367 - 113.
Ramli H, Zainal NFA, Hess M, Chan CH. Basic principle and good practices of rheology for polymers for teachers and beginners. Chemistry Teacher International. 2022; 4 (4):307-326 - 114.
Fang FF, Choi HJ, Choi WS. Two-layer coating with polymer and carbon nanotube on magnetic carbonyl iron particle and its magnetorheology. Colloid and Polymer Science. 2010; 288 (3):359-363 - 115.
Sedlačík M, Pavlínek V, Sáha P, Švrčinová P, Filip P, Stejskal J. Rheological properties of magnetorheological suspensions based on core–shell structured polyaniline-coated carbonyl iron particles. Smart Materials and Structures. 2010; 19 (11):115008 - 116.
Fang FF, Liu YD, Choi HJ, Seo Y. Core–shell structured carbonyl iron microspheres prepared via dual-step functionality coatings and their magnetorheological response. ACS Applied Materials & Interfaces. 2011; 3 (9):3487-3495 - 117.
Kwon SH, Sim B, Choi HJ. Magnetorheological characteristics of nano-sized iron oxide coated polyaniline composites. IEEE Transactions on Magnetics. 2016; 52 (7):1-4 - 118.
Kim JH, Fang FF, Choi HJ, Seo Y. Magnetic composites of conducting polyaniline/nano-sized magnetite and their magnetorheology. Materials Letters. 2008; 62 (17):2897-2899 - 119.
Piao SH, Bhaumik M, Maity A, Choi HJ. Polyaniline/Fe composite nanofiber added softmagnetic carbonyl iron microsphere suspension and its magnetorheology. Journal of Materials Chemistry C. 2015; 3 (8):1861-1868 - 120.
Moon IJ, Kim MW, Choi HJ, Kim N, You C-Y. Fabrication of dopamine grafted polyaniline/carbonyl iron core-shell typed microspheres and their magnetorheology. Colloids and Surfaces A: Physicochemical and Engineering Aspects. 2016; 500 :137-145 - 121.
Min TH, Choi HJ, Kim N-H, Park K, You C-Y. Effects of surface treatment on magnetic carbonyl iron/polyaniline microspheres and their magnetorheological study. Colloids and Surfaces A: Physicochemical and Engineering Aspects. 2017; 531 :48-55 - 122.
Kim JN, Dong YZ, Choi HJ. Pickering emulsion polymerized polyaniline/zinc-ferrite composite particles and their dual electrorheological and magnetorheological responses. ACS Omega. 2020; 5 (13):7675-7682 - 123.
Kim HM, Kang SH, Choi HJ. Polyaniline coated ZnFe2O4 microsphere and its electrorheological and magnetorheological response. Colloids and Surfaces A: Physicochemical and Engineering Aspects. 2021; 626 :127079 - 124.
Kim HM, Jeong JY, Kang SH, Jin H-J, Choi HJ. Dual electrorheological and magnetorheological behaviors of poly(N-methyl aniline) coated ZnFe2O4 composite particles. Materials. 2022; 15 (7):2677 - 125.
Kim TH, Choi HJ. Fabrication and shear response of conducting polymer-coated zinc ferrite particles under magnetic/electric field. IEEE Transactions on Magnetics. 2022; 58 (2):1-5 - 126.
Dong Y, Wang S, Choi HJ. Poly(N-methylaniline)/magnetite microsphere and its electrical and magnetic dual responses. Polymer. 2022; 240 :124492 - 127.
Jeong JY, Kim S, Baek E, You CY, Choi HJ. Suspension rheology of polyaniline coated manganese ferrite particles under electric/magnetic fields. Colloids and Surfaces A: Physicochemical and Engineering Aspects. 2023; 656 :130438 - 128.
Dong YZ, Choi K, Kwon SH, Nam J-D, Choi HJ. Nanoparticles functionalized by conducting polymers and their electrorheological and magnetorheological applications. Polymers. 2020; 12 (1):204