Electro-conducting properties and applications of polyaniline (PANI)-coated textiles.
Abstract
With the development of smart and functional textiles, electro-conductive fabrics based on polyaniline have attracted much attention due to its unique chemical structure, ease of preparation, flexibility, stability, excellent electrical conductivity, and sensing properties. As a result, polyaniline-based fabrics are widely used in various applications, including electromagnetic shielding, electronics, sensing, monitoring, and biomedicine. This chapter reviews the state-of-the-art technologies for fabricating polyaniline-coated woven, non-woven, and knitted fabrics based on natural and synthetic polymers, describing the fabrication methods, characterization techniques, and applications.
Keywords
- polyaniline-coated fabric
- polyaniline
- antibacterial textile
- electro-conductive fabric
- mechanical sensor
- ammonia gas sensor
- pH sensor
1. Introduction
Textiles are used daily in several applications, such as apparel, household textiles, furniture, medical and protective equipment, buildings, and vehicles. Textiles enable products’ functionalities and performance as well as esthetics and comfort. The contemporary textile industry is continuously challenged with innovative applications based on new technologies. Therefore, “smart textiles,” “E-textiles,” “functional textiles,” and “wearable electronic textiles” are among the terms used to represent the potential applications. Smart textiles have become the most promising next-generation wearable fabrics [1, 2, 3, 4, 5, 6, 7]. Smart textiles can respond to external physical and chemical stimuli, communicate by sensor technology, and adapt to changing surroundings [5, 6, 8]. The main aim behind developing smart textiles is to introduce new applications using efficient methodologies without compromising the intrinsic comfort, flexibility, and light weight of the fabric. Smart textiles have enormous potential in different applications possessing properties, including antibacterial, thermochromic (response to changes in temperature), shape-memory (response to external stimuli), hydrophobic and hydrophilic, oil-water separation, controlled release, drug delivery, photonics, sensors (response to pressure, pH, gas), antistatic, energy storage, and electro-conductive properties [1, 9, 10, 11, 12, 13, 14, 15, 16, 17].
Common textiles do not conduct electricity and are considered insulators. The textiles become electrically conductive by incorporating electro-conductive materials such as graphene (G), graphene oxide (GO), conductive nano, microparticles, and conjugated organic polymers on their surface for applications such as wearable electronic textiles [5, 6, 18, 19, 20]. The electrical conductivity of textiles depends on various factors, including the type and nature of the conductive coating as well as the ability to form an efficient interconnected conductive network within porous textile substrate. Incorporating conductive metallic particles often results in rigid fabrics with reduced flexibility that cannot maintain their functionality after washing. Therefore, flexible, deformable, stretchable, and durable electro-conductive threads are critical for electro-conductive textiles that serve as smart textiles for information transfer and computation capability while accommodating the movement of the human body [5, 6, 15, 21, 22, 23, 24, 25, 26]. For those reasons, intrinsic conductive polymers are the most suitable candidates for replacing conductive fillers to fabricate conductive textiles.
In recent decades, intrinsically conductive polymers (ICPs) have been extensively studied due to their unique electronic and electro-optical properties, and they are an essential part of the development of smart textiles [27, 28, 29]. In 1976, Shirakawa, MacDiarmid, and Heeger made a breakthrough discovery of a thin polyacetylene film oxidized with iodine vapor resulting in electrical conductivity [30]. They were awarded the Nobel Prize in Chemistry for discovering electrical conductivity in organic-conjugated materials in 2000 [31]. Over the years, dozens of ICPs have been introduced, among them polypyrrole (PPy), polyaniline (PANI), polythiophenes (PThs), and their derivatives. Their exceptional combination of properties, including electrical conductivity, electromagnetic shielding ability, light weight, flexibility, low cost, solution processability, good adhesion to diverse substrates, and ease of preparation, makes them ideal candidates for the development of smart textiles [21, 32, 33]. PANI is one of the most applied organic-conjugated polymers on textile due to its unique chemical structure, environmental stability, ease of preparation in aqueous solution, and excellent film-forming ability [34]. The electrical conductivity usually correlates to the molecular weight of the conjugated polymer, having reported that PANI with high molecular weight achieved higher electrical conductivity than the low molecular weight derivatives [35, 36, 37, 38]. The molecular structure of PANI consists of repeating units of the monomer aniline, as shown in Figure 1. The location of the N-atom between the phenyl rings enables the formation of various oxidation states, which significantly affect the physicochemical and mechanical properties of PANI [39]. There are three basic oxidation states of PANI: (a) leucoemeraldine (white/clear), (b) emeraldine (salt-green/base-blue), and (c) pernigraniline (blue/violet) [40, 41]. PANI exhibits two distinctive interconverting molecular forms in emeraldine oxidation state: salt (emeraldine salt (ES)) and base (emeraldine base (EB)). EB is a neutral form in which the amine groups are present as imines. Protonation of the imine nitrogen group with acid results in the doped ES state, in which the imines are converted into quaternary ammonium ions (Figure 2A) [42]. ES is the only conducting state among various forms of PANI that can be produced in an acidic solution (pH < 3.0) [43]. Doping with inorganic acids, such as hydrochloric or sulfuric acid, can influence the conductivity of PANI. As a result of doping, a charge transfer reaction occurs, which in turn creates active sites (polarons), adding an electron to the conduction band (n-doping) or removing an electron from the valence band (p-doping) [39]. The dopant degree can be easily changed by controlling the pH value of the dopant acid/base solution [44]. Both PANI-ES and PANI-EB display three ultraviolet-visible (UV-vis) absorption peaks. The absorption peaks between 255 and 286 nm and 328–349 nm are attributed to the π-π* transitions of the conjugated benzene rings of the PANI-ES and PANI-EB backbones. The third peak of PANI-ES located at 430–439 nm is due to polaron absorption resulting from the doping process. While the third band of PANI-EB is located at 610–650 nm, indicating the conversion of the imine nitrogen atoms of the benzenoid rings to quinonoid rings [45, 46, 47, 48, 49]. These unique properties of polyaniline make this remarkable material a suitable candidate for the development of chemical sensors [50], electromagnetic shielding devices [51], antibacterial protection [52], and electrochromic and electrochemical devices [53, 54] for smart textile applications.
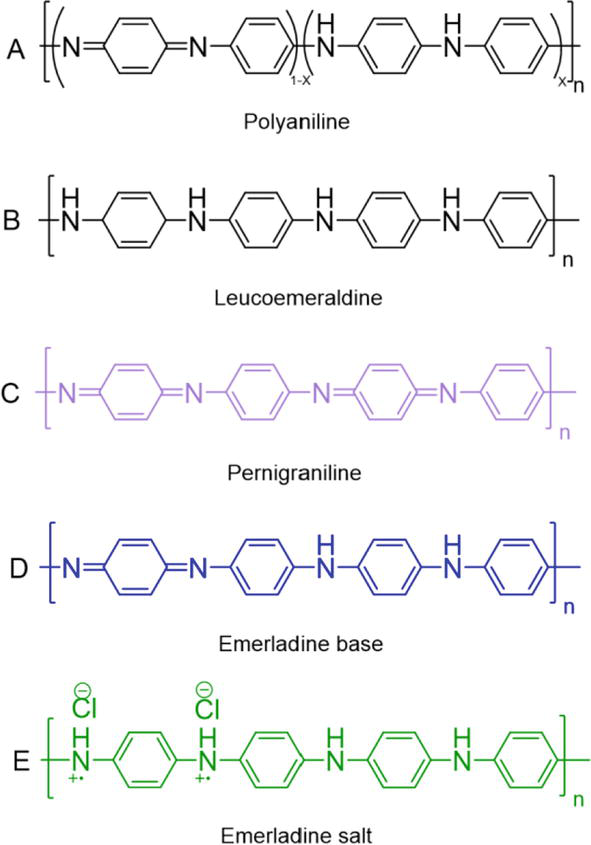
Figure 1.
Chemical structures of the different forms of polyaniline (PANI): (A) polyaniline, (B) leucoemeradine, (C) pernigraniline, (D) emeraldine base (EB), and (E) emeraldine salt (ES).
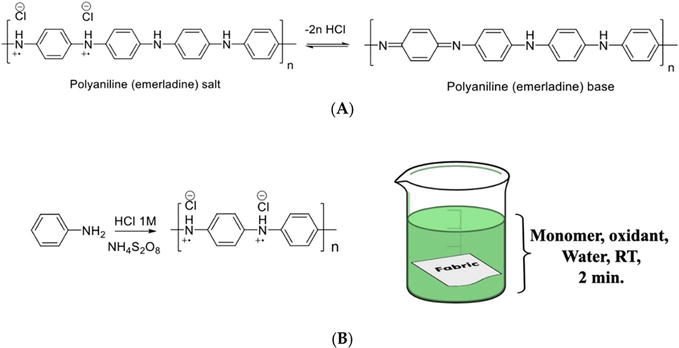
Figure 2.
Reversible transformation between polyaniline emeraldine salt (ES) and polyaniline emeraldine base (EB) (A), polymerization reaction of aniline, and schematic representation of fabric coating via in situ polymerization (B) [
2. Fabrication methods
Textiles are frequently coated with ICPs, such as a thin layer of PANI, to achieve electro-conductive and other functional properties. The common coating techniques are in situ polymerization, screen or inkjet printing, spray, and dip-coating [34]. The properties of the PANI-coated fabric depend on the aniline, oxidizing reagent, and dopant type and concentrations, the polymerization conditions, the pretreatment of the original fabric, and the type and style of the textile substrate.
2.1 In situ polymerization
Polyaniline can be polymerized directly on the textile surface via in situ polymerization of aniline monomer using an oxidizing reagent such as ammonium persulfate (APS) in an aqueous solution under acidic pH. During the polymerization process, the fabric is first immersed into an acidic aqueous solution containing aniline to ensure its absorption on the fabric surface for a specific time, followed by the introduction of APS triggering the polymerization reaction (Figure 2B). After the reaction is completed, the fabric is washed with water and organic solvents to remove the unreacted materials and byproducts, as shown in Figure 3 [42]. Depending on the type of fabric and the presence of reactive functional groups on the fabric surface, the growing polymer chains may be attached to the fabric via physical or chemical bonding [55]. The in situ method has been successfully applied on different textile substrates, such as cotton [56], poly(ethylene terephthalate) (PET) [57], nylon [58], polypropylene (PP) [59], silk [60], and more [61, 62, 63]. Over the years, it has become one of the most popular methods for developing multifunctional electro-conductive textiles [61]. For example, Sheibani et al. carried out in situ polymerization of PANI on cotton fabrics in the presence of hydrochloric acid (HCl) as a dopant and APS as an oxidant to achieve the electrical conductivity. The electrical resistivity of PANI-coated cotton fabrics was found to be strongly dependent on the monomer concentration and the molar ratio between aniline and oxidant. When using 0.019 mol of aniline and aniline-to-dopant molar ratio of 1:7, the electrical resistivity of the composite fabrics reached the level of 23 × 106 Ω/square, which was six orders of magnitude lower in comparison with the original cotton fabric [56].
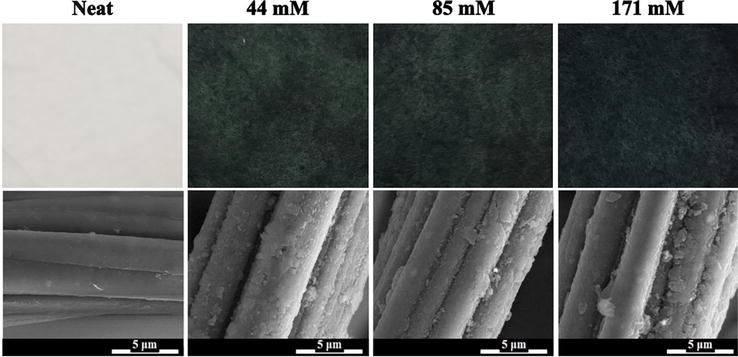
Figure 3.
Polyaniline (PANI)-coated poly(ethylene terephthalate) PET:viscose fabrics at different PANI concentrations and scanning electron microscopy (SEM) images of the fabric at 20,000× [
Wang et al. reported the coating of woven polyester fabric by in situ polymerization using p-toluenesulfonic acid (p-TSA) as a dopant. The coated fabrics showed good polarizing and absorbing-attenuation abilities for electromagnetic shielding applications when the concentrations of aniline, oxidant, and p-TSA were 0.4 mol/l, 0.4–0.5 mol/l, and 0.2 mol/l, respectively [64].
Another option is to utilize a two-step in situ polymerization. The first stage involves wetting of the fabric in an aniline solution for several minutes or hours to ensure efficient monomer absorption on the surface of the fabric. The second stage involves either immersion or spraying of the fabric with an aqueous/acidic solution containing oxidizing reagent. Kumar et al. and Lau et al. applied the two-step fabrication method to obtain a PANI layer on woven polypropylene and non-woven fabrics with ammonia gas-sensing properties [65, 66]. Most of the studies on the fabrication of PANI-based fabrics via in situ polymerization result in the formation of physical bonding between the polar chemical groups on the surface of the fabrics and the PANI chains. Covalent grafting of PANI onto the surface of fabrics is more challenging from a synthetic point of view; however, it may offer more accurate control of the surface properties and provide coating durability. Wu et al. reported a multistep process for covalent attachment of PANI to the surface of cotton fabrics. The fabrication method included the attachment of the polymeric graft chains containing epoxy functional end-groups to the hydroxyl groups of the cellulose, which were subsequently chemically linked to the 4-amino phenethylamine molecules via an amino-epoxy ring opening reaction. The modified fabric was then subjected to in situ polymerization, creating a PANI graft layer on the surface of the cotton fabric [67].
2.2 Printing techniques
2.2.1 Screen printing
Screen printing is a well-known, versatile, low-cost printing method based on high-viscosity inks forced and squeezed through the screen in a predetermined pattern mesh. This printing technique transfers ink through the mesh onto the fabric in the desired pattern [68, 69, 70]. Consequently, screen printing is widely used to fabricate smart textiles, realizing that depositing a pattern of functional ink onto fabric creates flexible and functional coatings. The inks and the substrates have a crucial role in screen printing technology, and their properties will determine the performance of the printed fabric [71]. There are various commercial inks commonly used based on plasticized polyvinyl chloride microparticles and Speedball®, which is based on poly(methyl methacrylate) particles. Screen printing has significant potential for manufacturing wearable electronics. It is one of the most cost-effective and efficient methods for producing electro-conductive patterned coatings on different fabrics. PANI-based inks for screen printing textiles have been extensively investigated [72]. For instance, Wang et al. developed flexible circuit patterns on woven polyester fabric. Conductive pastes containing G/PANI with different concentrations of aniline between 0 and 25 w/v% and viscosity range of 3000–25,000 Pa s were successfully prepared. The highest electrical resistivity of 0.6 × 103 Ω/square was achieved when the aniline concentration was 25 w/v%. The authors found that the electrical conductivity depends on the printed width line; as the width increases, the conductivity also increases; however, it was independent of the printing time [73]. In addition, Yu et al. prepared and printed a conductive nano-silver/PANI paste on cotton fabric to form conductive circuits for flexible electronic devices. PANI was found to enhance adhesion and prevent the self-assembly of the silver nanoparticles [74]. Ma et al. prepared thermo-conductive cotton fabric by incorporating eigenstate PANI with commercial ionic liquid (1-decyl-3-methylimidazolium bromide ([DMIm]Br)) via screen printing. Although the cotton surface was rough, the inks wrapped the cotton fibers uniformly. When the coated fabric was exposed to near-infrared (NIR) irradiation at 350 mW, the electrical current was increased by 150% [75].
Furthermore, the Gray group reported screen printing of a pH electrode on polyester fabric using commercial ink combined with PANI powder and dodecylbenzene sulfonic acid as a dopant [76]. In follow-up research, the authors fabricated the first screen-printed PANI composite sensor on a textile fabric that can be applied with textile-based microfluidic channels. The schematic illustration of screen printing is shown in Figure 4A, and a screen-printed PANI composite electrode is shown in Figure 4B [77].
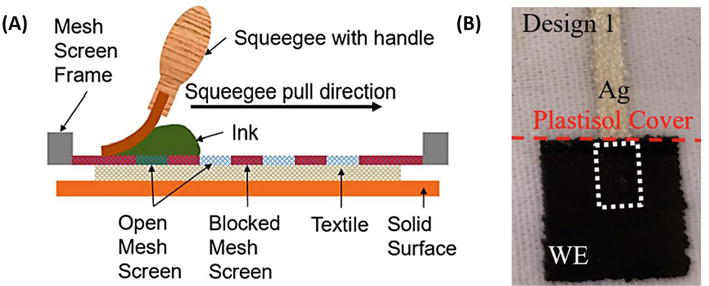
Figure 4.
(A) Schematic representation of typical textile screen printing; (B) polyaniline (PANI) composite screen printing on polyester fabric [
2.2.2 Inkjet printing
The inkjet printing method is attractive and versatile for controlled depositions of functional materials suitable for various surfaces. In inkjet printing, the structures are built up droplet by droplet on the surface of the substrate. Inkjet printing can be advantageous due to its low cost, esthetic finish, excellent resolution, and minimal material consumption. In comparison to screen printing, no mask is required, and there is flexibility in changing the printed pattern design depending on the final application. One of the requirements of this technique is to use low-viscosity fluids having viscosity values under 20 centiPoise (cP) and surface tension between 28 and 350 Nm−1 [78, 79]. Until today, this processing method has not been widely explored for PANI-coated fabrics, with only a limited number of studies reported in recent years. Stempien et al. proposed a facile method based on sequential line-by- line reactive inkjetting of aniline monomer and APS on polyacrylonitrile (PAN), cotton, PET, cotton/PET, wool, and cotton/wool fabrics for electromagnetic interference (EMI) shielding applications. The electro-conductive lines were printed on the surfaces of the fabrics by a selected-controlled pattern having the first nozzle print the aqueous solution of aniline hydrochloride and immediately after, the second nozzle prints the aqueous solution of ammonium persulfate or vice versa, as shown in Figure 5 [80, 81].
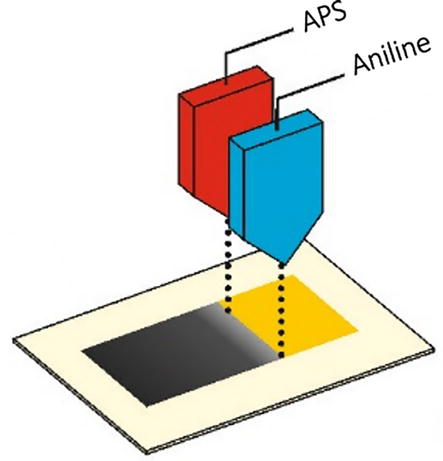
Figure 5.
Schematic representation of inkjet-printed textile of the polyaniline (PANI) layer [
2.3 Spray-coating
In the spray-coating technique, the fabric can be sprayed with aniline solution directly or polymerized by immersion in aniline solution, followed by spraying an APS solution. By using a screen mesh, one can control the pattern of the conductive particles or droplets on the textile substrate. Similar to screen-printing and inkjet-printing techniques, spray-coating has yet to be extensively investigated in fabricating PANI-based conductive textiles [79]. Qin et al. developed a multifunctional protective spray-coating based on a layer-by-layer assembly of PANI and GO on cotton fabrics. The GO particles were efficiently dispersed in the PANI nanowire arrays, affording nanoscale coating with controllable thickness and excellent antistatic, self-extinguishing, and antimicrobial properties [82]. Yu et al. also fabricated electrically conductive PANI/two-dimensional (2D) metal carbide (MXene)/cotton fabrics by a spray-coating method for acid/alkali-responsive and tunable electromagnetic interference (EMI) shielding applications, as shown in Figure 6 [51].
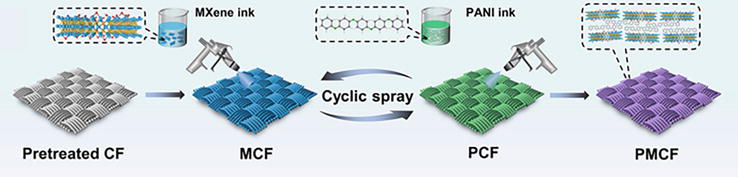
Figure 6.
Schematic representation of fabrication of electrically conductive PANI/MXene/cotton fabrics by spray coating [
2.4 Dip-coating
Dip-coating is a common approach for conductive textile materials due to its low cost, simplicity, and scale-up ability. This technique requires no specialized equipment, making it widely used [21]. The dip-coating method demonstrates a thin electro-conductive layer created on the surface of the fabric by immersion into an aqueous acidic solution containing PANI [83]. One of the drawbacks of this method is the formation of non-uniform coatings limiting the control of the coating thickness [84]. Various processing parameters, such as temperature, solution concentration, dip-coating time, and withdrawal speed, strongly impact the properties of the coating [83, 85]. There are several reports on the fabrication of PANI-coated fabrics via the dip-coating technique. Mahat et al. used dip-coating to fabricate a conductive polyaniline cotton fabric with phosphoric acid and p-TSA as dopants. The results revealed an increased thickness layer of PANI and improved electro-conductivity with increased dopant concentration [47]. The authors later described the fabrication of PANI-coated polyester fabric via dip-coatings that exhibited good antibacterial properties (Figure 7) [52]. Another study presented the preparation of thermo-electric polyester/linen woven by dip-coating into acetone suspension containing PANI/graphene nanosheets (GNSs). The preparation method included ultrasonication to establish efficient dispersion of GNS in acidic solution prior to the introduction of the aniline monomer and APS [86]. Wang et al. utilized dip-coating for the fabrication of a flexible gas sensor based on PANI and graphene nanoplatelets on PP fabric. In this case, the fabric was first dipped into graphene suspension, which was prepared via ultrasonication and addition of sodium dodecylbenzenesulfonate to enhance the graphene dispersion, followed by dipping into a PANI solution. The sensor fabric demonstrated a detection limit of 100 parts per billion (ppb) for ammonia gas [61]. Alamer et al. prepared a dip-coated PANI/carbon black (CB) composite on cotton fabric. Increasing PANI/CB concentration showed deeper coating penetration between the fibers [87].
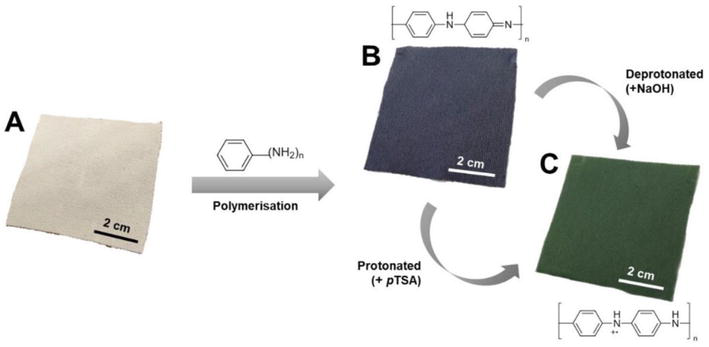
Figure 7.
Polyaniline (PANI) dip-coating process on polyester fabric [
3. Properties and applications
3.1 Electrical conductivity
Electrical conductivity is an important characteristic of PANI-coated textiles [88]. The electronic properties of PANI are associated with the emeraldine oxidation state and can be altered by reversible oxidation/reduction and protonation/deprotonation processes. The molecular weight and color of the PANI depend on the chemical composition of the reactants, concentration, and reaction conditions used for its synthesis. The parameters affecting the electrical conductivity of PANI-coated fabrics include the amount of polymer deposition, density, structure, and nature, which is evident due to a high number of picks per inch, resulting in lower surface resistivity [89, 90]. For example, Madhusoothanan et al. investigated the electrical resistivity of PANI/PET fabrics prepared via in situ polymerization with different weaving patterns of twill, satin, and plain, and 50–80 picks per inch. Their results revealed that among 80 picks per inch fabrics, the twill fabric showed lower surface resistivity of 1300 Ω/square compared to satin and plain fabrics that exhibited resistivity values of 1340 Ω/square and 1644 Ω/square, respectively. In addition, a reduction in surface resistivity was observed with increased fabric density for all weaving types [91]. Cellulose-based fabrics show efficient deposition of PANI due to the hydrogen bonds created between the aniline monomer and the surface of the yarns. This is reflected by a significant reduction in the electrical resistivity of the coated cotton-based fabrics compared to the untreated ones. For example, Hong et al. reported an electrical resistivity of 0.275 × 103 Ω cm obtained for knitted cotton fabric (255 grams per square meter (GSM)), which was eight orders of magnitude lower than the pristine fabric [92]. The concentration of aniline used for the polymerization also affects the electrical resistivity of the coated fabrics, which is decreased upon increasing the monomer concentration [42, 56, 93]. The type of dopant used for the polymerization of PANI may also affect the electrical properties of the fabrics. For example, using hydrochloric acid, hydrofluoric acid (HF), and hydrobromic acid (HBr) for aniline polymerization on cotton, wool, and polyester woven fabrics, the highest conductivity was observed for the bromine-doped PANI-coated fabrics [23]. A further enhancement in electrical conductivity of the PANI-coated fabrics may be achieved by combining PANI with carbon-based additives such as carbon black, carbon nanotubes, graphene, and graphene oxide, or conductive polymers such as polypyrrole and metal oxides such as MnO2 [40, 63, 87, 94, 95, 96, 97, 98, 99, 100]. To improve the interaction between PANI and the surface of the fabric to provide a good distribution of the conducting coating compositions, various compounds, such as polystyrene sulfonate (PSS) [101, 102], chitosan (CTS) [103], poly(2-methoxyaniline-5-sulfonic acid) (PMAS) [104], nanodiamond [20], polyetherimide [61], and 4-amino phenethylamine-glycidyl methacrylate [67], were also introduced. These materials contain polar chemical groups that establish efficient secondary interactions, such as hydrogen bonding with the aniline monomer, and effectively bridge between PANI molecules to form a continuous electro-conducting network. Table 1 summarizes the electrical properties of PANI-coated textiles with various coating compositions and their applications.
Textile type and coating composition | Fabrication method | Electrical conductivity/resistivity | Application | Ref. |
---|---|---|---|---|
PANI/cotton woven (143 GSM) | In situ polymerization, HCl, ammonium persulfate (APS) | 71 × 106 Ω/square | Electro-conductive textile | [56] |
PANI/silk crepe, PANI/reduced graphene oxide (RGO)/silk crepe | In situ polymerization, HCl, APS | 1.92 × 103 Ω/cm, 0.19 × 103 Ω/cm | Wearable electronic textile | [93] |
PANI/wool, PANI/PET, PANI/nylon 6, PANI/acrylics, PANI/cotton | In situ polymerization, HCl, potassium iodide (KI), APS, potassium bichromate | 104–106 Ω/square | Electro-conductive textile | [63] |
PANI/cotton knitted (255 GSM) | In situ polymerization, HCl, APS | 0.275 × 103 Ω·cm | Strain sensor | [92] |
PANI/nylon 6 woven | In situ polymerization, HCl, APS | 0.6 × 10−1 S/cm | Highly electro-conductive clothing | [105] |
PANI/PAN (220 GSM), PANI/cotton (120 GSM), PANI/wool (150 GSM), PANI/cotton/PET (50/50) (250 GSM), PANI/cotton/wool (25/75) (180 GSM), All woven fabrics | Reactive inkjet printing, water, APS | 233 Ω/square, 702 Ω/square, 1570 Ω/square, 1550 Ω/square,438 Ω/square | Electro-conductive textile in electromagnetic interference shielding | [81] |
PANI/PET, PANI/PET/viscose (70/30), PANI/bamboo, PANI/bamboo/cotton (70/30), All woven fabrics | In situ polymerization, HCl, APS | 29 Ω·m, 163.2 Ω·m, 12.4 Ω·m, 8.9 Ω·m, 6.2 Ω·m | Electro-conductive textiles | [90] |
PANI/viscose (170 GSM), PANI/PET (150 GSM), PANI/PET:viscose (50:50) (370 GSM), All non-woven fabrics | In situ polymerization, HCl, APS | 5.0 × 107 Ω/square, 10 × 106 Ω/square, 1.6 × 105 Ω/square | Electro-conductive and antibacterial textile | [42] |
PANI/cotton (192 GSM) woven | In situ polymerization, HCl, HF, and HBr, APS | 3281 μS/cm (HCl), 2071 μS/cm (HF) and 3314 μS/cm (HBr) | Electro-conductive textile | [23] |
PANI/4-aminophenethylamine-glycidyl methacrylate/cotton | Covalently grafting by multistep treatment process, APS | 1 × 107 Ω/square | Multifunctional electro-conductive textiles | [67] |
PANI/Ag/cotton (120 GSM) | In situ polymerization, aniline hydrochloride, APS | 19 × 106 Ω/square | Electrostimulation or monitoring of wound dressing | [106] |
PANI/MnO2/cotton | In situ polymerization, HCl, APS | 1.43 × 10−1 Ω−1·m−1 | Energy storage device | [99] |
PANI/CB/cotton | Dropcasting, dimethylsulfoxide (DMSO) | 0.494 × 103 Ω/square | Electro-conductive textile | [87] |
PANI/GO/cotton woven | In situ polymerization, HCl, APS | 48.35 Ω·cm | Electro-conductive and UV-blocking textile | [98] |
PPy/PANI/cotton woven (120 GSM) | In situ polymerization, aniline hydrochloride, APS | 210 Ω/square | Monitoring of carnivorous plant response | [97] |
PANI/ramie woven (70 GSM), PANI/PEI/ramie | In situ polymerization, HCl, APS | 16 × 106 Ω/square, 0.35 × 106 Ω/square | Electro-conductive multifunctional textiles | [61] |
PANI/carbon nanotubes (CNTs)/G/PET woven | In situ polymerization, H2SO4, APS | 114 Ω/square | Supercapacitor electrode textile | [100] |
PANI/chitosan/wool (80 GSM) | Two-step polymerizations, HCl, APS | 11.32 S/cm | Electro-conductive and antibacterial textile | [103] |
PANI/PMAS/wool-nylon-lycra | In situ polymerization, HCl, APS | 342 × 103 Ω/square | Strain sensor | [104] |
PANI/boron-doped nanocrystalline diamond (BDND)/wool knitted (180 GSM) | In situ polymerization, HCl, APS | 6.4 × 103 Ω/square | Wearable strain sensor | [20] |
PANI/polystyrene sulfonate (PSS)/polyamide (112 GSM), PANI/PSS/wool (184 GSM) | In situ polymerization, water, APS | 1.4 × 10−4 S/cm, 1.1 × 10−4 S/cm | Sensor-based textile | [101] |
PANI/PSS/nylon woven | Layer-by-layer (LBL) assembly, in situ polymerization, p-toluenesulfonic acid (p-TSA), APS | 1.7 × 10−5 S/cm | Electro-conductive textile | [102] |
PANI/polyester (plain, twill, satin) | In situ polymerization, HCl, APS | 1644 Ω/square, 1300 Ω/square, 1340 Ω/square | Electro-conductive textile | [91] |
Table 1.
3.2 Polyaniline-based textile sensors
Due to the unique chemical structure of PANI, it can act as a stimuli-responsive material, responding to changes in the environment by changing its color and electrical conductivity. Consequently, PANI-coated fabrics have been extensively studied as sensors detecting different chemicals, pressure, or mechanical changes in the environment [6, 26, 34, 107, 108].
3.2.1 Gas sensors
Polyaniline-coated fabrics are promising candidates for various gas-sensing applications due to the reversible acid/base reactions with gas molecules accompanied by the change in color and electrical conductivity [109, 110, 111]. Figure 8 shows a chemical reaction of a quaternary ammonium moiety of PANI-ES forming PANI-EB [110, 111, 112]. Extensive research has been focused on the development of PANI-coated fabrics as sensors for the detection and monitoring of ammonia, methanol, ethanol, acetone, hydrogen sulfide (H2S), nitrogen dioxide (NO2), and other gases [113, 114, 115]. Essential parameters for PANI-based gas sensors are the detection limit that defines the lowest concentration of the gas that can be reliably detected, and the “selectivity” refers to the ability to distinguish between various gases [116]. For instance, Jia et al. fabricated PANI on cotton fabric for detecting ammonia gas in the range of 1–20 parts per million (ppm), while the detection of H2S in the range between 1 and 1000 ppm was studied by de Souza et al. [117, 118]. The incorporation of additives, such as graphene, graphene oxide [50], and MXene [51], into PANI coating was shown to significantly improve the diffusion and penetration depth for the analyte gas molecules [110, 111, 119, 120, 121, 122, 123, 124]. Kim et al. fabricated a hybrid sensor based on PANI/G/PP, allowing the detection of ammonia gas from 100 ppb to 75 ppm with a detection limit of 1 ppm. When the sensor was exposed to 50 ppm of ammonia, the response and recovery times were 114 s and 23 s, respectively. The sensor also sensed H2S gas in the range of 10–50 ppm, with a response time of 138 s and a recovery time of 22 s for 50 ppm (Figure 9) [59]. Another gas sensor was fabricated by spraying PANI and multiwall carbon nanotubes (MWCNTs) on the PP fabric. The sensor was characterized for detecting ammonia in the 20–100 ppm range and demonstrated a detection limit of 200 ppb, as presented in Figure 10a. Furthermore, compared to nine other organic protic and aprotic solvents, the sensor showed more significant selectivity for the ammonia gas, as shown in Figure 10b [65]. Table 2 summarizes the composition, fabrication methods, and gas-sensing characteristics of the main reported textiles-based gas sensors.
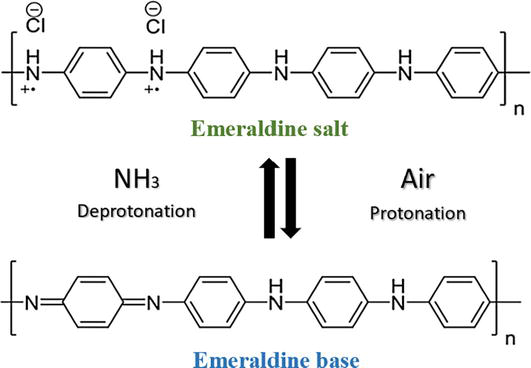
Figure 8.
Acid-base reaction between polyaniline (PANI) and ammonia (NH3).
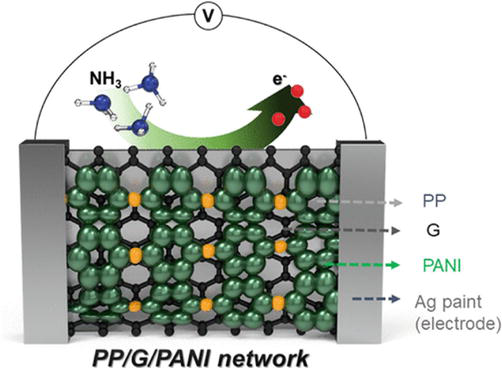
Figure 9.
Sensing mechanism of the PP/G/PANI hybrid sensor [
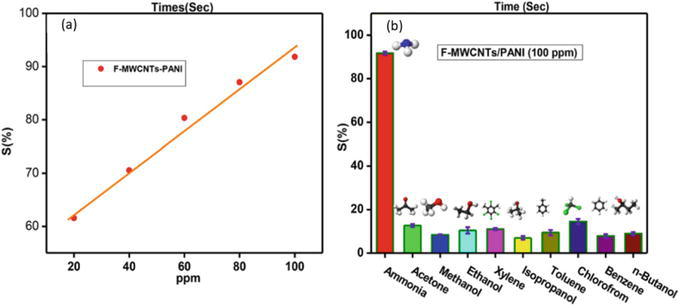
Figure 10.
Sensor response: (a) sensor response plot of F-MWCNTs/PANI toward the exposure of ammonia; (b) selectivity of the F-MWCNTs/PANI [
Sensor fabric | Fabrication method | Gas type | Detection range [ppm] | Response value [ppm] | Response time [sec] | Recovery time [sec] | Ref. |
---|---|---|---|---|---|---|---|
PANI/cotton | In situ polymerization | NH3 | 25–100 | — | — | — | [113] |
PANI/nylon 6 | In situ polymerization | NH3 | 1000 | — | — | — | [125] |
PANI/cotton | In situ polymerization | NH3 | 100 | 100 | 1.9 | 30 | [117] |
PANI/PP | Inkjet printing | NH3 | 15–100 | — | — | — | [114] |
PANI/PET | In situ polymerization | NH3 | 29 | — | — | — | [115] |
NO2 | 69 | ||||||
PANI/cotton | In situ polymerization | H2S | 1–1000 | — | — | — | [118] |
PANI/G/PP | In situ polymerization | NH3 | 0.01–75 | 50 | 114 | 23 | [59] |
H2S | 10–50 | 50 | 138 | 22 | |||
PANI/MWCNTs/PP | In situ polymerization | NH3 | 20–100 | 100 | 9 | 30 | [65] |
V2O5/PANI/GO/PET | In situ polymerization | NH3 | 0.5–100 | 20 | 70 | 520 | [50] |
1 | 78 | 259 | |||||
PANI/MXene/cotton | Spray-coating | NH3 | 10–200 | 50 | 308 | — | [51] |
PANI/WO3/cotton | In situ polymerization | NH3 | 3–150 | 100 | 122 | 165 | [119] |
PANI/clean wiper | In situ polymerization | NH3 | 10–320 | 20 | 50 | 500 | [120] |
Table 2.
Textile gas sensors based on polyaniline (PANI) coatings.
3.2.2 pH sensors
Textiles have become an attractive platform for fabricating pH sensors in the past decade due to their breathability, flexibility, and bending ability. Consequently, PANI-based textile sensors are proposed for monitoring skin pH for different skin conditions and diseases. Similar to the gas detection mechanism, depending on the pH values of the environment, the nitrogen atoms of the PANI backbone undergo protonation/deprotonation reactions between the imine groups and the quaternary ammonium ions. These processes allow rapid and reversible transformation between PANI-ES and PANI-EB in the pH range of 2–12 that are obtained by the changes of color, electrical and optical properties (Figures 8 and 11) [126, 127, 128, 129]. For example, Gray et al. fabricated a pH sensor based on screen printing of PANI-incorporated commercial ink on polyester fabric. The sensor properties were evaluated in the 3–10 pH range and showed a sensitivity of −28.2 mV/pH [76]. Other studies showed that PANI emeraldine salt had a better sensitivity of −42.6 mV/pH than PANI emeraldine base, which had a sensitivity of −27.9 mV/pH [77]. Koo et al. fabricated a skin pH sensor using a paste composed of carbon nanotubes (CNTs), agarose, and PANI on cotton, regular polyester, waterproof polyester, and thermoplastic polyurethane as an overlayer to improve stability. The sensor displayed a linear dependence on pH ranging from 5 to 9 with a 45.9 mV/pH sensitivity and maintained its sensing ability after repeated bending cycles [130]. Another wearable pH sensor was prepared from PANI combined with chopped carbon fibers and showed a Nernstian response of 58.0 mV/pH in the presence of pH values in the 4–8 range [131].
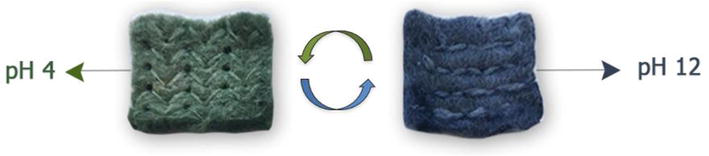
Figure 11.
Polyaniline (PANI)-coated non-woven cotton fabric functional as an optical pH sensor.
3.2.3 Mechanical sensors
Wearable sensors can interact with the human body/skin and monitor the movements of different body parts as well as physiological signals such as muscle vibrations and pulse [132, 133, 134]. Textile-based PANI sensors are divided into strain (triboelectric) and pressure (piezoelectric) sensors, responding to the corresponding stimuli by changing their electrical conductivity [132, 135]. Mechanical sensors can monitor stress, torque, pressure, or force applied to a textile [136, 137, 138]. Textile-based strain and pressure sensors show a good correlation between the applied mechanical deformation and the change in the electrical resistivity [139, 140, 141]. When strain is applied to the fabric, it causes the straightening of the yarns, increasing the density, improving the solid-state packing of the PANI molecular chains, and increasing the electrical conductivity of the coated fabric due to more efficient charge transport. Upon structural relaxation of the fabric, the free volume between PANI molecules increases, decreasing the electrical conductivity, as shown in Figure 12 [143, 144]. As a result, testing electrical conductivity changes as a function of the applied strain shows that the PANI-coated fabric sensors exhibit high strain linearity. The performance of the strain sensor depends on the weaving structure and intrinsic elasticity of the fabrics as well as the ability to form an efficient coating with strong interactions between the PANI chains and the fabric, enabling it to withstand tensile deformation. For example, Hong et al. developed a strain sensor based on PANI/cotton knitted fabric showing good repeatability for strain values up to 10% with excellent stretch recovery of 1000 cycles. When the strain was increased to 20%, the sensing repeatability was affected by the damaged PANI layer [92]. Kannaian et al. introduced a strain sensor based on PANI/nylon-lycra (92:8) fabric that exhibited changes in electrical conductivity up to 50% strain due to typical human body movement and bending angles up to 90o. Above these angles, they have yet to get a significant response. The gauge factor represents the sensitivity of a strain sensor, which was 0.92 [145]. Another PANI-based wearable strain sensor was fabricated using a 4-way stretch nylon-spandex (80:20) tricot fabric encapsulated with waterborne polyurethane coating to maintain the PANI onto the fabric. The sensor can monitor different knee states, such as walking and running, hand movements, and breathing rates in a strain range between 2.5 and 30%, with a gauge factor of 1–2.5 while detecting loadings up to 50 g. In addition, the sensor showed the ability to endure repeating 10% strain for 1000 cycles with a relative resistance change (ΔR/R) between 2.5 and 23% [146]. Another study described a strain sensor fabricated on lycra fabric using PANI/graphene nanoplatelets/silicon rubber successfully monitored up to 40% strain with a change of ΔR/R between 0 and 30%, and a gauge factor of 67.3. In addition, the sensor could monitor the bending angle of a finger up to 120° [147]. Composite coatings containing PANI and carbon nanotubes or titanium dioxide were fabricated on milk protein-based and knitted polyester fabrics operated at an even larger strain scale of 50–60% [62, 148]. Table 3 summarizes the composition, fabrication methods, and strain characteristics of the main reported textiles-based strain sensors.
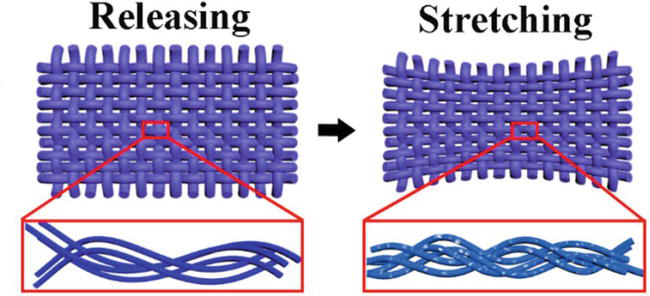
Figure 12.
Schematic illustration of the structural change in the conductive polymers (CPs)-coated polyester/spandex textile under a stretching force [
Sensor fabric | Fabrication method | Operating regime/sensing range [%] | Resistance | Cycling stability [cycles] | Monitoring ability | Ref. |
---|---|---|---|---|---|---|
PANI/cotton knitted | In situ polymerization | 0–20 | — | 1000 | Elbow, knee, finger, and, laryngeal | [92] |
PANI/nylon-lycra (92:8) | In situ polymerization | 0–60 | 12 ×103–11 × 103 Ω | 25 | Elbow and knee | [134] |
PANI/nylon-lycra (92:8) | In situ polymerization | 0–50 | 22 × 103–15 × 103 Ω | 5 | Elbow | [145] |
PANI/ nylon-spandex (80:20) | In situ polymerization | 0–25 | 5.1 × 103–3.8 × 103 Ω | 1000 | Knee and hand movement | [146] |
PANI/GNPs/SR/lycra | Spin-coating | 0–40 | 2.23 × 103–5 × 102 Ω | 40 | Finger | [147] |
PANI/TiO2/polyester | In situ polymerization | 0–60 | — | 100 | — | [148] |
PANI/CNTs/protein | In situ polymerization | 0.1–50 | — | — | Laryngeal, finger, elbow, and knee | [62] |
Table 3.
Textile strain sensors based on polyaniline (PANI) coatings.
Li et al. reported a pressure sensor made of PANI-coated non-woven cotton fabrics and screen-printed electrodes with an excellent sensitivity of 46.48 kPa−1 in a pressure range of up to 4.5 kPa. The sensor showed a low detection limit of 0.46 Pa, fast response and relaxation time of 7 and 16 ms, respectively, and high stability for more than 250 cycles. They tested the detection of physiological signals, such as wrist movement shown in Figure 13A, and the plot in Figure 13B demonstrates the change in current between 2.75 and 3.05 μA. Figure 13C presents the fabrication of silver paste electrodes by screen printing on neat and PANI-coated cotton placed together using a feather weight to create a ΔI/I change, as shown by a 3D graph in Figure 13D [149].
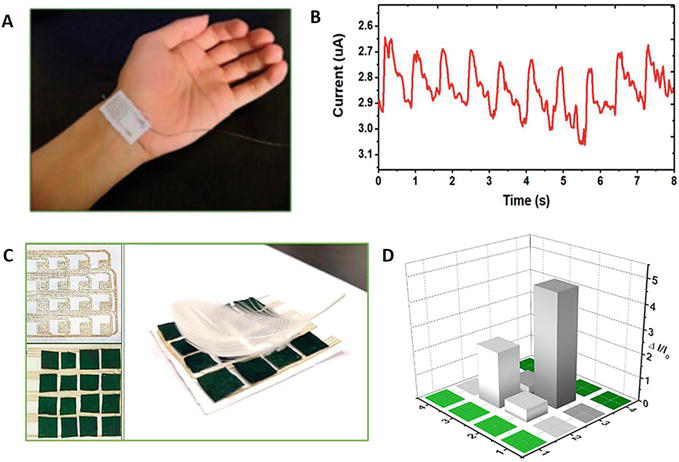
Figure 13.
(A) Real-time detection of physiological signals of a wrist by using the pressure sensor-based polyaniline (PANI). (B) Detection of a wrist pulse current. (C) A screen-printed sensor-based PANI and feather sensing. (D) By three-dimensional (3D) bar graph that displays the current change dependent on the pressure area [
Additionally, Zeng et al. designed a PANI-coated cotton sensor for monitoring finger pressing and fingertip motion with normalized current change between 0 and 4 and 0–6, respectively. The sensor senses between 300 Pa and 30 kPa, with a response time of 0.4 s and a relaxation time of 0.2 s [120]. Yu et al. prepared a PANI/Ag/PET pressure sensor with high sensitivity and good linearity for monitoring walking, running, squatting, and jumping. The sensor showed an immediate response at pressures between 2.5 and 6.5 kPa with a sharp increase in the relative change in the resistance (ΔR/R) due to an increase in the effective contact area [150]. Yu et al. prepared a PANI/nano-silver/cotton mechanical sensor. The sensor showed a sensitivity of 0.04–0.10 kPa−1 with a sensing range of 0–20 kPa, and a quick response and recovery time of 0.4 s. The sensor demonstrated 500 loading and unloading cycles with a resistance of 3.5 × 106 Ω and 1.1 × 106 Ω, respectively. Preparing a fabric with a layer-by-layer structure of PANI and nano-silver increases the electro-conductivity and contributes to excellent properties. This sensor can effectively monitor the movements of vocal cord vibrations, arm, elbow, and foot [151]. Table 4 summarizes the composition, fabrication methods, and pressure characteristics of the main reported textiles-based pressure sensors.
Sensor fabric | Fabrication method | Pressure range | (I-I0)/I | (R-R0/R) | Response time/Recovery time | Compression force and frequency for cycling stability measurement | Cycling stability | Monitoring ability | Ref. |
---|---|---|---|---|---|---|---|---|---|
PANI/cotton | In situ polymerization | 0.5–30 N | — | — | — | 5 N and 5 Hz | 2000 | Leaning back, sitting, and hand | [133] |
PANI/cotton | In situ polymerization | 4–30 kPa | 0.5–16 | — | 0.4 s/0.2 s | 6 kPa and 0.5 Hz | 1000 | Fingers | [120] |
PANI/cotton | In situ polymerization | 0–30 kPa | 0–800 | — | 7 ms/16 ms (2 kPa) | 5 kPa and 0.05 Hz | 250 | Wrist and neck | [149] |
PANI/Ag/PET | In situ polymerization | 2.5–9 kPa | — | 2.5–65 | 0.3 s/0.2 s (9 kPa) | 9 kPa | 1300 | Walking, running, squatting, and jumping | [150] |
PANI/nano-silver/cotton | Printing | 0–8 N | — | 0–60 | 0.4 s/0.4 s | — | 500 | Vocal cord, elbow, wrist, and foot | [151] |
Table 4.
Textile pressure sensors based on polyaniline (PANI) coatings.
3.3 Electromagnetic interference shielding
Electromagnetic interference shielding can protect electronic equipment and humans against external electromagnetic irradiation. EMI shielding is based on three components: reflection, absorption, and multiple reflections inside the shielding material at different frequency ranges [152]. Textiles are useful for protecting materials due to their light weight, flexibility, and drapability. Textile-based EMI shielding is strongly driven by using new materials, such as ICPs, to fabricate protective coatings [153, 154]. In this emerging field, PANI-based textiles have gained considerable research interest due to their tunable electronic properties combined with the ease of processability and the ability to control the thickness and the amount of shielding coating. PANI can be used solely or in combination with other conjugated polymers, metal particles, or carbon-based additives. EMI shielding properties are also affected by other factors, such as the fabric structure, porosity, and thickness.
For example, Ozdemir et al. reported an average electromagnetic shielding efficiency of 3.8 dB and an average absorption value of 48% for PANI-coated cotton fabrics [155]. Also, Sun produced a PANI-coated cotton fabric with a low surface resistivity of 0.5 kΩ and electromagnetic shielding effectiveness of up to 60%. They demonstrated the correlation between the oxidant concentration and the shielding effectiveness, resulting in a decreased electromagnetic shielding effectiveness when increasing the APS concentration [156]. In addition, Jia et al. prepared PANI-coated cotton composite strips with ammonia gas-sensing and EMI shielding properties. The EMI shielding effectiveness varied depending on the frequency and increased to about −9 dB in the frequency range of 4.5 GHz and 9 GHz. [117]. Muthukumar et al. investigated the correlation between the fabric type and the EMI shielding effectiveness. They displayed cotton, polyester, and nylon PANI-coated fabrics in the frequency range of 8–12 GHz with EMI values of −1.62, −2.78, and −1.5 dB, respectively [157]. In another study, Kannaian et al. developed a conductive PANI-coated nylon-lycra fabric with an EMI shielding efficiency of 26 dB in the frequency range of 8–12 GHz [158]. Dhawan et al. fabricated PANI coating on polyester fabric with various PANI layer thicknesses, showing a strong correlation between the coating thickness layer and the shielding effectiveness. The highest shielding effectiveness of 21 dB was obtained for a three-layered PANI-coated polyester fabric, while a PANI-coated silica fabric exhibited a shielding effectiveness of 35 dB at 101 GHz [159]. The EMI shielding can be enhanced by fabricating PANI composite coatings with other conductive materials. For example, Yu et al. used a spray-coating layer-by-layer assembly to prepare a conductive PANI/MXene/cotton fabric with an EMI shielding effectiveness of 54 dB, compared to 45 dB, which was obtained for an MXene/cotton fabric shown in Figure 14. The researchers found a direct relationship between the number of spray cycles and EMI shielding properties. It was shown that acid/alkali environments can trigger EMI shielding. When treated with an alkali gas, the shielding effectiveness decreased to 15 dB, while exposure to acidic gas increased the EMI shielding effectiveness to 22 dB [51]. Yu et al. reported a PANI/nickel-tungsten-phosphorus (Ni-W-P) coating on functional polyimide fabric with an electrical resistivity of 0.08 Ω/square and an outstanding EMI shielding effectiveness of 103 dB. This study highlights the relationship between high electrical conductivity and excellent shielding performance [58]. Additionally, Sebastian et al. incorporated graphite to enhance the shielding properties of PANI nanofibers due to their conductivity and flake-like morphology. They tested the PANI powder and the PANI/graphite powder to show the effect of graphite additive on the electrical conductivity and shielding effectiveness. The results showed increased electrical conductivity and shielding effectiveness from 18.5 S/cm to 24 S/cm and from 71 to 77 dB to 83–89 dB, respectively. Incorporating PANI nanofibers/graphite composite coating with a thickness of about 0.1 mm into cotton and nylon fabrics resulted in 11–15 dB shielding effectiveness in the frequency range of 8.2–18 GHz [160]. Another study described PANI/silver-plated PET fabrics with surface resistivity of 0.1 Ω/square, with shielding effectiveness between 60 and 90 dB in the frequency range between 30 kHz and 3 GHz, which was barely affected after ultrasonic washing for 30 min (50–90 dB) [57]. Table 5 summarizes the composition, fabrication methods, and shielding effectiveness characteristics of the main reported textiles-based EMI shielding.
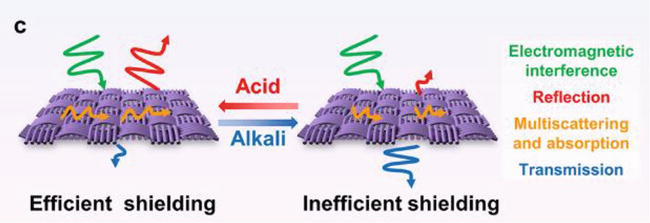
Figure 14.
Schematic draw of acid/alkali-responsive and tunable EMI shielding behavior of the PANI/MXene/cotton fabric [
Sensor fabric | Fabrication method | Electrical conductivity/resistivity | Frequency range | EMI shielding effectiveness | Ref. |
---|---|---|---|---|---|
PANI/cotton | In situ polymerization | 350 Ω | 6–14 GHz | 3.8 dB | [155] |
PANI/cotton | In situ polymerization | 0.5 × 103 Ω | 1–1500 MHz | 60% | [156] |
PANI/cotton | In situ polymerization | — | 0.3–9000 MHz | 0–(−9) dB | [117] |
PANI/MXene/cotton | Spray-coating | 0.64 Ω/square | 8.2–12.4 GHz | ∼54 dB | [51] |
PANI/cotton, PANI/polyester, and PANI/nylon | In situ polymerization | 7 × 103 Ω/square, 5 × 103 Ω/square, 5 × 103 Ω/square | 8–12 GHz | −1.62, −2.78, and − 1.5 dB | [157] |
PANI/nylon-lycra (92:8) | In situ polymerization | 3.5 × 103 Ω/square | 8–12 GHz | 26.38 dB | [158] |
PANI /polyester and PANI /silica | In situ polymerization | 26 Ω·cm, and 20–28 Ω ·cm | 101 GHz | 21.48 dB and 35.61 dB | [159] |
PANI/artificial suede | In situ polymerization | 9.2 S/m | 8.2–12.4 GHz | 25.90 dB | [161] |
PANI/nickel-tungsten-phosphorus/polyimide | In situ polymerization | 0.08 Ω/square | 0.3–3000 MHz | 103 dB | [58] |
PANI/graphite/cotton and PANI/graphite/nylon | In situ polymerization | — | 8.2–18 GHz | 15 dB and 14 dB | [160] |
PANI/silver-plated/PET | In situ polymerization | 0.1 Ω/square | 0.3–3000 MHz | 50–90 dB | [57] |
Table 5.
Polyaniline (PANI)-based fabrics with electromagnetic interference (EMI) shielding properties.
3.4 Antibacterial textile
Over the past decade, extensive research has been focused on developing textiles that can either kill or inhibit the proliferation of bacteria, fungi, and viruses [162]. Microorganisms can easily multiply on certain types of fabrics, causing odors, hygiene problems, and a reduction in the mechanical strength of the fabric. Furthermore, the increased spreading rate of epidemics brought the need for antibacterial fabrics for their use in public places and personal care. Consequently, dozens of studies have been introduced describing the incorporation of various antibacterial agents into fabrics, including nanoparticles of silver, zinc, and magnesium oxides, essential oils, chitosan, and chitin [42, 162, 163, 164, 165, 166, 167]. The exact mechanism of the antibacterial effect of these and other reagents is unclear. However, the one proposed mechanism involves electrostatic interactions between cationic moieties of the antibacterial reagent and negatively charged surface or RNA/DNA proteins of the bacterial membrane [42, 52, 168, 169]. Emeraldine salt of polyaniline is an excellent material for antibacterial applications since it may be considered a poly quaternary ammonium ion containing many effective cationic sites within a single polymer chain, as shown in Figure 15 [42]. In addition, PANI is insoluble in water and can establish strong intermolecular interactions with the fabric providing coating durability, unlike other organic quaternary ammonium salt-based reagents and N-halamines that are water soluble and can be removed upon repeated washings.
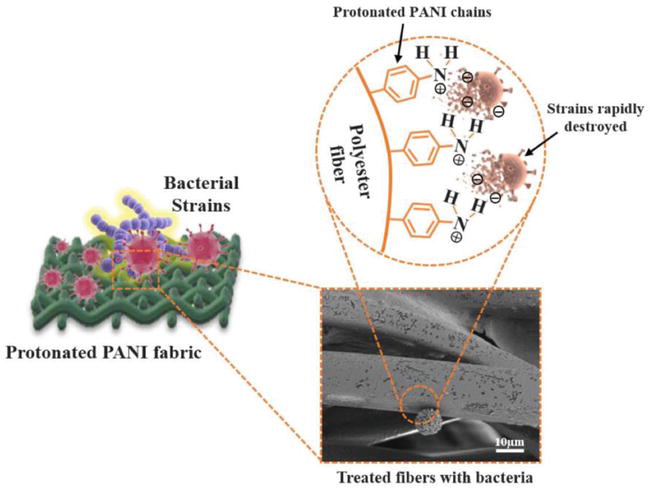
Figure 15.
Schematic representation of protonated polyaniline (PANI)-integrated fabric when exposed to the bacteria [
Furthermore, a large quantity of small molecule-based antibacterial reagents will be required to achieve the effect compared to a small quantity of PANI, which can be sufficient due to its polymeric nature. The transformation of PANI-ES and PANI-EB is accompanied by a color change from green to blue, which can provide a visual indication of the antibacterial effectiveness and the possibility of restoration by re-doping. The antibacterial properties of PANI-coated cotton fabric were first reported by Bhat et al. [170]. Since then, a significant number of publications have been published describing PANI-based fabrics with antimicrobial effects against a variety of Gram-negative and Gram-positive bacteria, including
Antibacterial fabric | Fabrication method | Test method | Bacteria type | Antibacterial efficiency | Ref. |
---|---|---|---|---|---|
PANI/viscose, PANI/PET, and PANI/PET:viscose (50:50) | In situ polymerization | Colony count | 100% | [42] | |
100%, 99.9997%, and 99.65% | |||||
PANI/cotton | In situ polymerization | Colony count | 95% | [170] | |
85% | |||||
92% | |||||
PANI/cotton and PANI/polyester | Dip-coating | Kirby-Bauer disc diffusion | 3.5 ± 0.170 mm and 10.3 ± 0.048 mm | [85] | |
0 mm and 3.5 ± 0.170 mm | |||||
0 mm and 5.0 ± 0.140 mm | |||||
PANI/polyester | Dip-coating | Kirby-Bauer disc diffusion | 22.30 ± 0.03 mm | [52] | |
21.50 ± 0.09 mm | |||||
19.11 ± 0.02 mm | |||||
24.33 ± 0.02 mm | |||||
14.12 ± 0.07 mm | |||||
21.35 ± 0.08 mm | |||||
PANI/GO/cotton | Spray-coating | Kirby-Bauer disc diffusion | 10.2 mm | [82] | |
11.0 mm | |||||
PANI/carbon nitride (CN)/cotton | In situ polymerization | Colony count | 72.6% | [171] | |
PANI/Ag/cotton | In situ polymerization | Kirby-Bauer disc diffusion | 3 mm | [106] | |
5 mm | |||||
PANI/silk and PANI/copper sulfide nanoparticles (CuSNPs)/silk | In situ polymerization | Colony count | 45.3% and 99.64% | [60] | |
∼90% and 99.99% | |||||
PANI/wool and PANI/chitosan/wool | Two-step polymerization | Colony count | 64.92% and 99.99% | [103] | |
69.27% and 100% |
Table 6.
Antibacterial fabrics based on polyaniline (PANI).
Amir’s group applied PANI on non-woven fabrics, including viscose, PET, and PET:viscose (50:50), and counted the bacteria by a Colony count method that estimates the number of bacteria on the plate. The PET, viscose, and 50:50 PET:viscose PANI-coated fabrics exhibited a 100% antibacterial effect on the
4. Conclusions and future outlook
The increasing number of publications describing fabrication and properties of PANI-based fabrics reflects the tremendous potential of this exceptional material for a broad range of smart textile applications. Its processability from aqueous solutions is a significant feature behind exploring different coating and printing techniques toward the realization of large-scale production. In situ polymerization method is one of the most studied coating techniques that was extensively studied, providing several solutions for thickness and quality control of PANI coating on different types of fabrics. The results of the published studies indicate that the electrical conductivity of PANI-coated textiles can be modified within a wide range by controlling the polymerization conditions, performing the coating method, and using additional conductive fillers. This allows one to adjust the electrical and other properties of the coated fabrics based on the target application requirements. The unique combination of reversible stretchability of the textile yarns and PANI molecular chains is a key feature behind the pressure and strain-sensing ability of PANI-coated fabrics. Despite much of the progress that has been achieved in this field, future research should aim to increase the robustness of the coatings by establishing strong adhesion between the PANI coating and the fabric allowing multiple deformations of the fabric without damaging the PANI layer. The reported high values of EMI shielding effectiveness, combined with the intrinsic flexibility and drapability of the fabrics, indicate a strong potential of PANI-coated textile for protecting applications. The chemical structure of PANI is fundamental to the fabrication of smart textile sensors that respond to external stimuli by changing the electrical conductivity and optical properties. Furthermore, the antibacterial properties, gas- and pH-sensing abilities of PANI-coated fabrics can be reversible due to the rapid transformation between ES and EB forms. PANI establishes strong hydrogen bond interactions with cellulose-based fabrics containing hydroxyl groups on the surface, providing long-term coating stability. Nevertheless, the adhesion of PANI layer is weaker for low surface energy fabrics, such as polyester or nylon. Despite the several strategies introduced to improve coating adhesion by incorporating intermediate layers with polar chemical groups, further research is required to develop durable, functional coatings with high washing stability.
Overall, the research studies reviewed in this chapter provide a solid platform for the future development of PANI-coated fabrics with advanced and multifunctional properties and commercial realization.
References
- 1.
Dan Y, Buzhor M, Raichman D, Menashe E, Rachmani O, Amir E. Covalent surface functionalization of nonwoven fabrics with controlled hydrophobicity, water absorption, and pH regulation properties. Journal of Applied Polymer Science. 2020; 138 (6):1-11. DOI: 10.1002/app.49820 - 2.
Xu F et al. 3D arch-structured and machine-knitted triboelectric fabrics as self-powered strain sensors of smart textiles. Nano Energy. 2023; 109 :1-10. DOI: 10.1016/j.nanoen.2023.108312 - 3.
Lorusso E, Ali W, Hildebrandt M, Mayer-Gall T, Gutmann JS. Hydrogel functionalized polyester fabrics by UV-induced photopolymerization. Polymers (Basel). 2019; 11 (8):1-10. DOI: 10.3390/polym11081329 - 4.
Goswami S et al. Human-motion interactive energy harvester based on polyaniline functionalized textile fibers following metal/polymer mechano-responsive charge transfer mechanism. Nano Energy. 2019; 60 :794-801. DOI: 10.1016/j.nanoen.2019.04.012 - 5.
Ramlow H, Andrade KL, Immich APS. Smart textiles: An overview of recent progress on chromic textiles. Journal of the Textile Institute. 2021; 112 (1):152-171. DOI: 10.1080/00405000.2020.1785071 - 6.
Shah MA, Pirzada BM, Price G, Shibiru AL, Qurashi A. Applications of nanotechnology in smart textile industry: A critical review. Journal of Advanced Research. 2022; 38 :55-75. DOI: 10.1016/j.jare.2022.01.008 - 7.
Xue P, Tao X, Leung MY, Zhang H. Electromechanical properties of conductive fibres, yarns and fabrics. Wearable Electronics and Photonics. 2005; 2005 :81-104. DOI: 10.1533/9781845690441.81 - 8.
Júnior HLO, Neves RM, Monticeli FM, Dall Agnol L. Smart fabric textiles: Recent advances and challenges. Text. 2022; 2 (4):582-605. DOI: 10.3390/textiles2040034 - 9.
Dan Y, Popowski Y, Buzhor M, Menashe E, Rachmani O, Amir E. Covalent surface modification of cellulose-based textiles for oil-water separation applications. Industrial and Engineering Chemistry Research. 2020; 59 (13):5456-5465. DOI: 10.1021/acs.iecr.9b05785 - 10.
Hu J. Controlled release of hydrogel modified textile products. Journal of Controlled Release. 2011; 152 (Suppl):e31-e33. DOI: 10.1016/j.jconrel.2011.08.104 - 11.
Subbiah DK, Babu KJ, Das A, Rayappan JBB. NiOx nanoflower modified cotton fabric for UV filter and gas sensing applications. ACS Applied Materials & Interfaces. 2019; 11 (22):20045-20055. DOI: 10.1021/acsami.9b04682 - 12.
Hartman C, Popowski Y, Raichman D, Amir E. Biodegradable polymer coating for controlled release of hydrophobic functional molecules from cotton fabrics. Journal of Coating Technology and Research. 2020; 17 (3):669-679. DOI: 10.1007/s11998-019-00278-3 - 13.
Mocioiu AM, Mocioiu OC. Intelligent textile with doped polyaniline. Macromolecular Symposia. 2021; 396 (1):10-12. DOI: 10.1002/masy.202000331 - 14.
Hashemikia S, Hemmatinejad N, Ahmadi E, Montazer M. A novel cotton fabric with anti-bacterial and drug delivery properties using SBA-15-NH2/polysiloxane hybrid containing tetracycline. Materials Science and Engineering C. 2016; 59 :429-437. DOI: 10.1016/j.msec.2015.09.092 - 15.
Civan L, Kurama S. A review: Preparation of functionalised materials/smart fabrics that exhibit thermochromic behaviour. Materials Science and Technology. 2021; 37 (18):1405-1420. DOI: 10.1080/02670836.2021.2015844 - 16.
Wu Y et al. Long-term antibacterial protected cotton fabric coating by controlled release of chlorhexidine gluconate from halloysite nanotubes. RSC Advances. 2017; 7 (31):18917-18925. DOI: 10.1039/c7ra01464c - 17.
Ballottin D et al. Antimicrobial textiles: Biogenic silver nanoparticles against Candida and Xanthomonas. Materials Science and Engineering C. 2017; 75 :582-589. DOI: 10.1016/j.msec.2017.02.110 - 18.
Ojstršek A et al. Metallisation of textiles and protection of conductive layers: An overview of application techniques. Sensors. 2021; 21 (10):3508. DOI: 10.3390/s21103508 - 19.
Kazani I et al. About the collinear four-point probe technique’s inability to measure the resistivity of anisotropic electroconductive fabrics. Textile Research Journal. 2013; 83 (15):1587-1593. DOI: 10.1177/0040517512452951 - 20.
Rehman A, Houshyar S, Reineck P, Padhye R, Wang X, Houshyar S. Multifunctional smart fabrics through nanodiamond-polyaniline nanocomposites. ACS Applied Polymer Materials. 2020; 2 (11):4848-4855. DOI: 10.1021/acsapm.0c00789 - 21.
Ojstršek A, Jug L, Plohl O. A review of electro conductive textiles utilizing the dip-coating technique: Their functionality, durability and sustainability. Polymers (Basel). 2022; 14 (21):4713. DOI: 10.3390/polym14214713 - 22.
Pattanarat K, Petchsang N, Osotchan T, Tang IM, Kim YH, Jaisutti R. High conductivity and durability textile gas sensor-based polyaniline-decorated-poly(3,4-ethylenedioxythiophene)/poly(4-styrenesulfonate) for ammonia detection. ACS Applied Polymer Materials. 2022; 4 (12):9006-9014. DOI: 10.1021/acsapm.2c01374 - 23.
Teli M, Dash S, Desai P. Polyaniline based conductive textiles. Journal of The Institution of Engineers (India): Series E. 2014; 95 (2):75-79. DOI: 10.1007/s40034-014-0037-x - 24.
Abu Sadat MS, Teay SH, Shahariar H, Fink PL, Albarbar A. Review on smart electro-clothing systems (SeCSs). Sensors. 2020; 20 (587):1-23. DOI: 10.3390/s20030587 - 25.
Terse-Thakoor T et al. Thread-based multiplexed sensor patch for real-time sweat monitoring. npj Flexible Electronics. 2020; 4 (1):1-10. DOI: 10.1038/s41528-020-00081-w - 26.
Krifa M. Electrically conductive textile materials—Application in flexible sensors and antennas. Text. 2021; 1 (2):239-257. DOI: 10.3390/textiles1020012 - 27.
Moretti C, Tao X, Koncar V, Koehl L. A study on electrical performances and lifetime of a flexible electrochromic textile device. Autex Research Journal. 2014; 14 (2):76-81. DOI: 10.2478/aut-2014-0003 - 28.
Huang G, Liu L, Wang R, Zhang J, Sun X, Peng H. Smart color-changing textile with high contrast based on a single-sided conductive fabric. Journal of Materials Chemistry C Materials. 2016; 4 (32):7589-7594. DOI: 10.1039/c6tc02051h - 29.
Wei Y, Wang X, Torah R, Tudor J. Dispenser printing of electrochromic display on textiles for creative applications. Electronics Letters. 2017; 53 (12):779-781. DOI: 10.1049/el.2017.0119 - 30.
Shirakawa H, Louis EJ, MacDiarmid AG, Chiang CK, Heeger AJ. Synthesis of electrically conducting organic polymers: Halogen derivatives of polyacetylene, (CH)x. Journal of the Chemical Society, Chemical Communications. 1977; 16 :578-580. DOI: 10.1039/C39770000578 - 31.
Abu-Thabit NY. Chemical oxidative polymerization of polyaniline: A practical approach for preparation of smart conductive textiles. Journal of Chemical Education. 2016; 93 (9):1606-1611. DOI: 10.1021/acs.jchemed.6b00060 - 32.
Nezakati T, Seifalian A, Tan A, Seifalian AM. Conductive polymers: Opportunities and challenges in biomedical applications. Chemical Reviews. 2018; 118 (14):6766-6843. DOI: 10.1021/acs.chemrev.6b00275 - 33.
Cohen David N et al. Electro-conductive fabrics based on dip coating of cotton in poly(3-hexylthiophene). Polymers for Advanced Technologies. 2017; 28 (5):583-589. DOI: 10.1002/pat.3857 - 34.
Islam GMN, Ali A, Collie S. Textile sensors for wearable applications: A comprehensive review. Cellulose. 2020; 27 (11):6103-6131. DOI: 10.1007/s10570-020-03215-5 - 35.
Stejskal J, Riede A, Hlavat D, Proke J, Helmstedt M, Holler P. The effect of polymerization temperature on molecular weight, crystallinity, and electrical conductivity of polyaniline. Synthetic Metal. 1998; 96 :55-61 - 36.
Yılmaz F, Küçükyavuz Z. The influence of polymerization temperature on structure and properties of polyaniline. E-Polymers. 2009; 5 :1-10 - 37.
Adams PN, Bowman D, Brown L, Yang D, Mattes BR. Molecular weight dependence of the physical properties of protonated polyaniline films and fibers. SPIE Smart Structures and Materials + Nondestructive Evaluation and Health Monitoring. 2001; 4329 :475-481. DOI: 10.1117/12.432679 - 38.
Macdiarmid AG, Epstein AJ. Polyaniline: Interrelationships between molecular weight, morphology, Donnan potential and conductivity. MRS Proceedings. 1992; 247 (1):565-576. DOI: 10.1557/PROC-247-565/METRICS - 39.
Rai R, Roether JA, Boccaccini AR. Polyaniline based polymers in tissue engineering applications: A review. Progress in Biomedical Engineering. 2022; 4 :1-38. DOI: DOI 10.1088/2516-1091/ac93d3 - 40.
Beygisangchin M, Rashid SA, Shafie S, Sadrolhosseini AR. Preparations, Properties, and applications of polyaniline and polyaniline thin films—A review. Polymers (Basel). 2021; 13 :1-46 - 41.
Bhadra S, Khastgir D, Singha NK, Lee JH. Progress in preparation, processing and applications of polyaniline. Progress in Polymer Science (Oxford). 2009; 34 (8):783-810. DOI: 10.1016/j.progpolymsci.2009.04.003 - 42.
Jarach N et al. Hybrid antibacterial and electro-conductive coating for textiles based on cationic conjugated polymer. Polymers (Basel). 2020; 12 (7):1-15. DOI: 10.3390/polym12071517 - 43.
Shoaie N et al. Electrochemical sensors and biosensors based on the use of polyaniline and its nanocomposites: A review on recent advances. Microchimica Acta. 2019; 186 (7):465. DOI: 10.1007/s00604-019-3588-1 - 44.
Tissera ND, Wijesena RN, Rathnayake S, de Silva RM, de Silva KMN. Heterogeneous in situ polymerization of polyaniline (PANI) nanofibers on cotton textiles: Improved electrical conductivity, electrical switching, and tuning properties. Carbohydrates Polymers. 2018; 186 :35-44. DOI: 10.1016/j.carbpol.2018.01.027 - 45.
Akbar SA, Satria E. Uv-vis study on polyaniline degradation at different phs and the potential application for acid-base indicator. Rasayan Journal of Chemistry. 2019; 12 (3):1212-1218. DOI: 10.31788/RJC.2019.1235370 - 46.
Gul S, Shah AUHA, Bilal S. Synthesis and characterization of processable polyaniline salts. Journal of Physics Conference Series. 2013; 439 (1):1-10. DOI: 10.1088/1742-6596/439/1/012002 - 47.
Roslan NC et al. Morphological and conductivity studies of polyaniline fabric doped phosphoric acid. Malaysian Journal of Analytical Sciences. 2020; 24 (5):698-706 - 48.
Paschoalin RT, Steffens C, Manzoli A, Paris EC, Herrmann PSP. PANI conductivity: A dependence of the chemical synthesis temperature. Macromolecular Symposia. 2012; 319 (1):48-53. DOI: 10.1002/masy.201100242 - 49.
Stejskal J, Kratochvíl P, Radhakrishnan N. Polyaniline dispersions 2. UV-Vis absorption spectra. Synthetic Metals. 1993; 61 (3):225-231. DOI: 10.1016/0379-6779(93)91266-5 - 50.
Xing X et al. Twistable and tailorable V2O5/PANI/GO nanocomposites textile for wearable ammonia sensing. Sensors Actuators B Chemie. 2022; 351 :130944. DOI: 10.1016/j.snb.2021.130944 - 51.
Li DY et al. Functional polyaniline/MXene/cotton fabrics with acid/alkali-responsive and tunable electromagnetic interference shielding performances. ACS Applied Materials & Interfaces. 2022; 14 (10):12703-12712. DOI: 10.1021/acsami.2c00797 - 52.
Aizamddin MF et al. Antibacterial performance of protonated polyaniline-integrated polyester fabrics. Polymers (Basel). 2022; 14 (13):1-17. DOI: 10.3390/polym14132617 - 53.
Gicevicius M, Cechanaviciute IA, Ramanavicius A. Electrochromic textile composites based on polyaniline-coated metallized conductive fabrics. Journal of the Electrochemical Society. 2020; 167 (15):155515. DOI: 10.1149/1945-7111/abb0f3 - 54.
Parvez MS, Rahman MM, Samykano M, Yeakub Ali M. Electrochemical characterization and joule heating performance of polyaniline incorporated cotton fabric. Physics and Chemistry of the Earth; 129 :103323. DOI: 10.1016/j.pce.2022.103323 - 55.
Allison L, Hoxie S, Andrew TL. Towards seamlessly-integrated textile electronics: Methods to coat fabrics and fibers with conducting polymers for electronic applications. Chemical Communications. 2017; 53 (53):7182-7193. DOI: 10.1039/c7cc02592k - 56.
Barani H, Miri A, Sheibani H. Comparative study of electrically conductive cotton fabric prepared through the in situ synthesis of different conductive materials. Cellulose. 2021; 28 (10):6629-6649. DOI: 10.1007/s10570-021-03928-1 - 57.
Mu S, Xie H, Wang W, Yu D. Electroless silver plating on PET fabric initiated by in situ reduction of polyaniline. Applied Surface Science. 2015; 353 :608-614. DOI: 10.1016/j.apsusc.2015.06.126 - 58.
Ding X, Wang W, Wang Y, Xu R, Yu D. High-performance flexible electromagnetic shielding polyimide fabric prepared by nickel-tungsten-phosphorus electroless plating. Journal of Alloys and Compounds. 2019; 777 :1265-1273. DOI: 10.1016/j.jallcom.2018.11.120 - 59.
Wu G et al. Polyaniline/graphene-functionalized flexible waste mask sensors for ammonia and volatile sulfur compound monitoring. ACS Applied Materials & Interfaces. 2022; 14 :56056-56064. DOI: 10.1021/acsami.2c15443 - 60.
Ren Y et al. Multifunctional textile constructed via polyaniline-mediated copper sulfide nanoparticle growth for rapid photothermal antibacterial and antioxidation applications. ACS Applied Nano Materials. 2022; 6 :1212-1223. DOI: 10.1021/acsanm.2c04797 - 61.
Ke G, Chowdhury MH, Jin X, Li W. Fabrication and properties of polyaniline/ramie composite fabric based on in situ polymerization. Polymers and Polymer Composites. 2021; 29 :S914-S925. DOI: 10.1177/09673911211028398 - 62.
Wang Y, Zhang X, Cao J, Huang X, Zhang X. Multifunctional E-Textiles Based on Biological Phytic Acid-Doped Polyaniline/Protein Fabric Nanocomposites. Advanced Materials Technology. 2021; 6 (6):1-8. DOI: 10.1002/admt.202100003 - 63.
Hirase R, Shikata T, Shirai M. Selective formation of polyaniline on wool by chemical polymerization, using potassium iodate. Synthetic Metals. 2004; 146 (1):73-77. DOI: 10.1016/j.synthmet.2004.06.009 - 64.
Zhao X, Sun J, Liu Y, Wang Y. The preparation and performance of polyaniline coated polyester fabrics. Journal of the Textile Institute. 2021; 112 (10):1700-1707. DOI: 10.1080/00405000.2020.1839217 - 65.
Maity D, Kumar RTR. Polyaniline anchored MWCNTs on fabric for high performance wearable ammonia sensor. ACS Sensors. 2018; 3 (9):1822-1830. DOI: 10.1021/acssensors.8b00589 - 66.
Qi J, Xu X, Liu X, Lau KT. Fabrication of textile based conductometric polyaniline gas sensor. Sens Actuators B Chem. 2014; 202 :732-740. DOI: 10.1016/j.snb.2014.05.138 - 67.
Wu B et al. Electrical switchability and dry-wash durability of conductive textiles. Scientific Reports. 2015; 5 :1-9. DOI: 10.1038/srep11255 - 68.
Agarwala S. Enabling new possibilities in smart textiles through printed electronics. In: 2019 IEEE 9th International Nanoelectronics Conferences, INEC 2019. 2019. pp. 1-6. DOI: 10.1109/INEC.2019.8853853 - 69.
Ali AE, Jeoti V, Stojanović GM. Fabric based printed-distributed battery for wearable e-textiles: A review. Science and Technology of Advanced Materials. 2021; 22 (1):772-793. DOI: 10.1080/14686996.2021.1962203 - 70.
Stoppa M, Chiolerio A. Wearable electronics and smart textiles: A critical review. Sensors (Switzerland). 2014; 14 (7):11957-11992. DOI: 10.3390/s140711957 - 71.
Gong X, Huang K, Wu YH, Zhang XS. Recent progress on screen-printed flexible sensors for human health monitoring. Sens Actuators A Physics. 2022; 345 :1-19. DOI: 10.1016/j.sna.2022.113821 - 72.
Roshni SB, Jayakrishnan MP, Mohanan P, Surendran KP. Design and fabrication of an E-shaped wearable textile antenna on PVB-coated hydrophobic polyester fabric. Smart Materials and Structures. 2017; 26 (10):1-8. DOI: 10.1088/1361-665X/aa7c40 - 73.
Zhu J, Song W, Peng J, Yin Y, Xu B, Wang C. Microwave thermally expanded graphene/polyaniline conductive paste for elaborate conductive pattern and conductive polyester fabric fabrication via screen printing. Journal of Coating Technology and Research. 2022; 19 (2):477-485. DOI: 10.1007/s11998-021-00530-9 - 74.
Wang Z, Wang W, Jiang Z, Yu D. Low temperature sintering nano-silver conductive ink printed on cotton fabric as printed electronics. Progress in Organic Coating. 2016; 101 :604-611. DOI: 10.1016/j.porgcoat.2016.08.019 - 75.
Zhang X, Wang Y, Fu D, Wang G, Wei H, Ma N. Photo-thermal converting polyaniline/ionic liquid inks for screen printing highly-sensitive flexible uncontacted thermal sensors. European Polymer Journal. 2021; 147 :1-8. DOI: 10.1016/j.eurpolymj.2021.110305 - 76.
Laffitte Y, Gray BL. Real-Time potentiometric pH-sensor using a screen-printable polyaniline composite on textiles. In: IEEE International Conference on Flexible and Printable Sensors and Systems. 2021. pp. 19-22. DOI: 10.1109/FLEPS51544.2021.9469705 - 77.
Laffitte Y, Gray BL. Potentiometric pH sensor based on flexible screen-printable polyaniline composite for textile-based microfluidic applications. Micromachines (Basel). 2022; 13 (9):1-19. DOI: 10.3390/mi13091376 - 78.
Loffredo F, Burrasca G, Quercia L, Della Sala D. Gas sensor devices obtained by ink-jet printing of polyaniline suspensions. Macromolecular Symposia. 2007; 247 :357-363. DOI: 10.1002/masy.200750141 - 79.
Tseghai GB, Malengier B, Fante KA, Nigusse AB, Van Langenhove L. Integration of conductive materials with textile structures, an overview. Sensors (Switzerland). 2020; 20 (23):1-28. DOI: 10.3390/s20236910 - 80.
Stempien Z et al. Inkjet printing of polypyrrole electroconductive layers based on direct inks freezing and their use in textile solid-state supercapacitors. Materials. 2021; 14 (13):1-20. DOI: 10.3390/ma14133577 - 81.
Stempien Z, Rybicki T, Rybicki E, Kozanecki M, Szynkowska MI. In-situ deposition of polyaniline and polypyrrole electroconductive layers on textile surfaces by the reactive ink-jet printing technique. Synthetic Metals. 2015; 202 :49-62. DOI: 10.1016/j.synthmet.2015.01.027 - 82.
Zeng F, Qin Z, Chen Y, Shan X. Constructing polyaniline nanowire arrays as efficient traps on graphene sheets to promote compound synergetic effect in the assembled coating for multifunctional protective cotton fabrics. Chemical Engineering Journal. 2021; 426 (February):130819. DOI: 10.1016/j.cej.2021.130819 - 83.
Tang X, Yan X. Dip-coating for fibrous materials: Mechanism, methods and applications. Journal of Solgel Science and Technology. 2016; 81 (2):378-404. DOI: 10.1007/s10971-016-4197-7 - 84.
Zeng W, Shu L, Li Q, Chen S, Wang F, Tao XM. Fiber-based wearable electronics: A review of materials, fabrication, devices, and applications. Advanced Materials. 2014; 26 :5310-5336. DOI: 10.1002/adma.201400633 - 85.
Omar SNI et al. Electrically conductive fabric coated with polyaniline: Physicochemical characterisation and antibacterial assessment. Emergent Mater. 2020; 3 (4):469-477. DOI: 10.1007/s42247-019-00062-4 - 86.
Amirabad R, Ramazani Saadatabadi A, Siadati MH. Preparation of polyaniline/graphene coated wearable thermoelectric fabric using ultrasonic-assisted dip-coating method. Materials Renewable Sustainable Energy. 2020; 9 (4):1-12. DOI: 10.1007/s40243-020-00181-7 - 87.
Alamer FA. Structural and electrical properties of conductive cotton fabrics coated with the composite polyaniline/carbon black. Cellulose. 2018; 25 (3):2075-2082. DOI: 10.1007/s10570-018-1667-9 - 88.
Elmogahzy YE. Performance characteristics of technical textiles: Part I: E-textiles. Engineering Text. 2020; 2020 :347-364. DOI: 10.1016/b978-0-08-102488-1.00014-9 - 89.
Pomfret SJ, Adams PN, Comfort NP, Monkman AP. Electrical and mechanical properties of polyaniline fibres produced by a one-step wet spinning process. Polymer (Guildf). 2000; 41 (6):2265-2269. DOI: 10.1016/S0032-3861(99)00365-1 - 90.
Perumalraj R. Electrical surface resistivity of polyaniline coated woven fabrics. Journal of Textiles Science and Engineering. 2015; 05 (03):1-5. DOI: 10.4172/2165-8064.1000196 - 91.
Neelakandan R, Madhusoothanan M. Electrical resistivity studies on polyaniline coated polyester fabrics. Journal of Engineering and Fiber Fabrication. 2010; 5 (3):25-29. DOI: 10.1177/155892501000500304 - 92.
Zhou X, Hu C, Lin X, Han X, Zhao X, Hong J. Polyaniline-coated cotton knitted fabric for body motion monitoring. Sensor Actuators A Physica. 2021; 321 :112591. DOI: 10.1016/j.sna.2021.112591 - 93.
Cao J, Wang C. Highly conductive and flexible silk fabric via electrostatic self assemble between reduced graphene oxide and polyaniline. Organic Electronics. 2018; 55 (January):26-34. DOI: 10.1016/j.orgel.2017.12.016 - 94.
Goswami S, Nandy S, Fortunato E, Martins R. Polyaniline and its composites engineering: A class of multifunctional smart energy materials. Journal of Solid State Chemical. 2023; 317 (July):123679. DOI: 10.1016/j.jssc.2022.123679 - 95.
Liu Y, Zhao X. The preparation and performance of a polyaniline/graphene composite coated fabric. Journal of the Textile Institute. 2021; 112 (8):1258-1265. DOI: 10.1080/00405000.2020.1809908 - 96.
Cheedarala RK, Parvez AN, Ahn KK. Electric impulse spring-assisted contact separation mode triboelectric nanogenerator fabricated from polyaniline emeraldine salt and woven carbon fibers. Nano Energy. 2018; 53 (May):362-372. DOI: 10.1016/j.nanoen.2018.08.066 - 97.
Bajgar V et al. Cotton fabric coated with conducting polymers and its application in monitoring of carnivorous plant response. Sensors. 2016; 16 (4):498. DOI: 10.3390/s16040498 - 98.
Tang X et al. Functionalization of cotton fabric with graphene oxide nanosheet and polyaniline for conductive and UV blocking properties. Synthetic Metals. 2015; 202 :82-88. DOI: 10.1016/j.synthmet.2015.01.017 - 99.
Attia RM, Yousif NM, Helal RH, Ali NM. Fabricating electronic textile using nano MnO2/polyaniline composites for capacitor device. Journal of Industrial Textiles. 2022; 51 (7):1161-1180. DOI: 10.1177/1528083719896765 - 100.
Jin LN et al. High-performance textile supercapacitor electrode materials enhanced with three-dimensional carbon nanotubes/graphene conductive network and in situ polymerized polyaniline. Electrochimica Acta. 2017; 249 :387-394. DOI: 10.1016/j.electacta.2017.08.035 - 101.
Mocioiu AM, Tudor IA, Mocioiu OC. Application of polyaniline for flexible semiconductors. Coatings. 2021; 11 (1):1-10. DOI: 10.3390/coatings11010049 - 102.
He J, Li R, Gu F. Preparation of polyaniline/nylon conducting fabric by layer-by-layer assembly method. Journal of Applied Polymer Science. 2012; 128 (3):1673-1679. DOI: 10.1002/app.38331 - 103.
Yu J, Pang Z, Zhang J, Zhou H, Wei Q. Conductivity and antibacterial properties of wool fabrics finished by polyaniline/chitosan. Colloids and Surfaces A: Physicochemical and Engineering Aspects. 2018; 548 :117-124. DOI: 10.1016/j.colsurfa.2018.03.065 - 104.
Wu J, Zhou D, Looney MG, Waters PJ, Wallace GG, Too CO. A molecular template approach to integration of polyaniline into textiles. Synthetic Metals. 2009; 159 (12):1135-1140. DOI: 10.1016/j.synthmet.2009.01.054 - 105.
Oh KW, Hong KH, Kim SH. Electrically conductive textiles by in situ polymerization of aniline. Journal of Applied Polymer Science. 1999; 74 (8):2094-2101. DOI: 10.1002/(SICI)1097-4628(19991121)74:8<2094::AID-APP26>3.0.CO;2-9 - 106.
Maráková N et al. Antimicrobial activity and cytotoxicity of cotton fabric coated with conducting polymers, polyaniline or polypyrrole, and with deposited silver nanoparticles. Applied Surface Science. 2017; 396 :169-176. DOI: 10.1016/j.apsusc.2016.11.024 - 107.
Castano LM, Flatau AB. Smart fabric sensors and e-textile technologies: A review. Smart Materials and Structures. 2014; 23 (5):1-27. DOI: 10.1088/0964-1726/23/5/053001 - 108.
Guo L, Bashir T, Bresky E, Persson NK. Electroconductive textiles and textile-based electromechanical sensors-integration in as an approach for smart textiles. In: Vladan K, editor. Smart Textiles and Their Applications. UK: Woodhead Publishing, Elsevier Ltd; 2016. pp. 657-693. DOI: 10.1016/B978-0-08-100574-3.00028-X - 109.
Bag A, Lee NE. Recent advancements in development of wearable gas sensors. Advanced Materials Technology. 2021; 6 (3):1-37. DOI: 10.1002/ADMT.202000883 - 110.
Fratoddi I, Venditti I, Cametti C, Russo MV. Chemiresistive polyaniline-based gas sensors: A mini review. Sensors Actuators B Chemical. 2015; 220 :534-548. DOI: 10.1016/J.SNB.2015.05.107 - 111.
Alrammouz R, Podlecki J, Abboud P, Sorli B, Habchi R. A review on flexible gas sensors: From materials to devices. Sensors Actuators A Physics. 2018; 284 :209-231. DOI: 10.1016/J.SNA.2018.10.036 - 112.
Kukla AL, Shirshov YM, Piletsky SA. Ammonia sensors based on sensitive polyaniline films. Sensors Actuators B Chemical. 1996; 37 (3):135-140. DOI: 10.1016/S0925-4005(97)80128-1 - 113.
Myat Swe M, Eamsa-Ard T, Kerdcharoen T. Fabrication of polyaniline coated conductive cotton for ammonia gas detection. MATEC Web of Conferences. 2018; 192 (02036):1-4. DOI: 10.1051/MATECCONF/201819202036 - 114.
Stempien Z et al. Ammonia gas sensors ink-jet printed on textile substrates. IEEE Sensors. 2017; 2017 :2-4. DOI: 10.1109/ICSENS.2016.7808457 - 115.
Collins GE, Buckley LJ. Conductive polymer-coated fabrics for chemical sensing. Synthetic Metals. 1996; 78 (2):93-101. DOI: 10.1016/0379-6779(96)80108-1 - 116.
Vivaldi F et al. Recent advances in optical, electrochemical and field effect pH sensors. Chem. 2021; 9 (2):1-17. DOI: 10.3390/chemosensors9020033 - 117.
Zhang W et al. Mechanical, electromagnetic shielding and gas sensing properties of flexible cotton fiber/polyaniline composites. Composites Science and Technology. 2020; 188 :1-7. DOI: 10.1016/j.compscitech.2019.107966 - 118.
de Almeida TM, da Silveira Maranhão F, de Carvalho FV, Middea A, de Araujo JR, de Souza Júnior FG. H2S sensing material based on cotton fabrics modified with polyaniline. Macromolecular Symposia. 2018; 381 (1):1-10. DOI: 10.1002/masy.201800111 - 119.
He M, Xie L, Luo G, Li Z, Wright J, Zhu Z. Flexible fabric gas sensors based on PANI/WO3 p−n heterojunction for high performance NH3 detection at room temperature. Science China Materials. 2020; 63 (10):2028-2039. DOI: 10.1007/s40843-020-1364-4 - 120.
Tang X et al. A low-cost polyaniline@textile-based multifunctional sensor for simultaneously detecting tactile and olfactory stimuli. Macromolecular Materials and Engineering. 2018; 303 (12):1-6. DOI: 10.1002/mame.201800340 - 121.
P. Cavallo et al. , “Functionalized polyanilines made by nucleophilic addition reaction, applied in gas sensors field,” Synthetic Metals, vol. 215, pp. 127–133, May 2016, doi: 10.1016/J.SYNTHMET.2016.02.013. - 122.
Cavallo P, Acevedo DF, Fuertes MC, Soler-Illia GJAA, Barbero CA. Understanding the sensing mechanism of polyaniline resistive sensors. Effect of humidity on sensing of organic volatiles. Sensors Actuators B Chemical. 2015; 210 :574-580. DOI: 10.1016/j.snb.2015.01.029 - 123.
Bai H, Shi G. Gas sensors based on conducting polymers. Sensors. 2007; 7 :267-307. DOI: 10.3390/s7030267 - 124.
Li ZF, Blum FD, Bertino MF, Kim CS. Understanding the response of nanostructured polyaniline gas sensors. Sensor Actuators B Chemical. 2013; 183 :419-427. DOI: 10.1016/j.snb.2013.03.125 - 125.
Hong KH, Oh KW, Kang TJ. Polyaniline-Nylon 6 composite fabric for ammonia gas sensor. Journal of Applied Polymer Science. 2004; 92 (1):37-42. DOI: 10.1002/app.13633 - 126.
Adhikari B, Majumdar S. Polymers in sensor applications. Progress in Polymer Science (Oxford). 2004; 29 (7):699-766. DOI: 10.1016/j.progpolymsci.2004.03.002 - 127.
Tang Y et al. Recent advances in wearable potentiometric pH sensors. Membranes (Basel). 2022; 12 (5):1-20. DOI: 10.3390/membranes12050504 - 128.
Hou X, Zhou Y, Liu Y, Wang L, Wang J. Coaxial electrospun flexible PANI//PU fibers as highly sensitive pH wearable sensor. Journal of Materials Science. 2020; 55 (33):16033-16047. DOI: 10.1007/s10853-020-05110-7 - 129.
Lindfors T, Ivaska A. pH sensitivity of polyaniline and its substituted derivatives. Journal of Electroanalytical Chemistry. 2002; 531 (1):43-52. DOI: 10.1016/S0022-0728(02)01005-7 - 130.
Choi MY et al. A fully textile-based skin pH sensor. Journal of Industrial Textiles. 2022; 51 :441S-457S. DOI: 10.1177/15280837211073361 - 131.
Guinovart T, Valdés-Ramírez G, Windmiller JR, Andrade FJ, Wang J. Bandage-based wearable potentiometric sensor for monitoring wound pH. Electroanalysis. 2014; 26 (6):1345-1353. DOI: 10.1002/elan.201300558 - 132.
Wu S. An overview of hierarchical design of textile-based sensor in wearable electronics. Crystals (Basel). 2022; 12 (555):1-32. DOI: 10.3390/cryst12040555 - 133.
Dudem B, Mule AR, Patnam HR, Yu JS. Wearable and durable triboelectric nanogenerators via polyaniline coated cotton textiles as a movement sensor and self-powered system. Nano Energy. 2019; 55 :305-315. DOI: 10.1016/j.nanoen.2018.10.074 - 134.
Muthukumar N, Thilagavathi G, Kannaian T. Analysis of piezoresistive behavior of polyaniline-coated nylon Lycra fabrics for elbow angle measurement. Journal of the Textile Institute. 2017; 108 (2):233-238. DOI: 10.1080/00405000.2016.1161696 - 135.
Zhou Z et al. Textile-based mechanical sensors: A review. Materials. 2021; 14 (20):1-22. DOI: 10.3390/ma14206073 - 136.
Callister W. Materials Science and Engineering: An Introduction. 7th ed. New York: John Wiley & Sons; 2007:131-166 - 137.
Beer FP, Russell Johnston JE, Dewolf JT, Mazurek DF. Mechanics of Materials. 6th ed. New York, NY: McGraw-Hill. 2006 - 138.
Soloman S. Sensors Handbook. 1999 - 139.
Gonçalves C, da Silva AF, Gomes J, Simoes R. Wearable e-textile technologies: A review on sensors, actuators and control elements. Inventions. 2018; 3 (14):1-13. DOI: 10.3390/inventions3010014 - 140.
Qin QH. Advanced Mechanics of Piezoelectricity 2013. Berlin, Heidelberg: Springer Jointly published with Higher Education Press; DOI: 10.1007/978-3-642-29767-0 - 141.
Manbachi A, Cobbold RSC. Development and application of piezoelectric materials for ultrasound generation and detection. Ultrasound. 2011; 19 (4):187-196. DOI: 10.1258/ult.2011.011027 - 142.
Chen X, Li B, Qiao Y, Lu Z. Preparing polypyrrole-coated stretchable textile via low-temperature interfacial polymerization for highly sensitive strain sensor. Micromachines (Basel). 2019; 10 (11):788. DOI: 10.3390/mi10110788 - 143.
Wu J, Zhou D, Too CO, Wallace GG. Conducting polymer coated lycra. Synthetic Metals. 2005; 155 :698-701. DOI: 10.1016/j.synthmet.2005.08.032 - 144.
Fan Q, Zhang X, Qin Z. Preparation of polyaniline/polyurethane fibers and their piezoresistive property. Journal of Macromolecular Science, Part B: Physics. 2012; 51 (4):736-746. DOI: 10.1080/00222348.2011.609795 - 145.
Muthukumar N, Thilagavathi G, Kannaian T. Design and development of polyaniline-coated fabric strain sensor for goniometry applications. International Journal of Science and Engineering Applications. 2013; 7560 :38-42. DOI: 10.7753/ijseancrtam.1010 - 146.
Rashid IA et al. Highly resilient and responsive fabric strain sensors: Their effective integration into textiles and wireless communication for wearable applications. Sensor Actuators A Physics. 2022; 346 :1-11. DOI: 10.1016/j.sna.2022.113836 - 147.
Huang Y, Gao L, Zhao Y, Guo X, Liu C, Liu P. Highly flexible fabric strain sensor based on graphene nanoplatelet–polyaniline nanocomposites for human gesture recognition. Journal of Applied Polymer Science. 2017; 134 (39):1-8. DOI: 10.1002/app.45340 - 148.
Tang X et al. Water-repellent flexible fabric strain sensor based on polyaniline/titanium dioxide-coated knit polyester fabric. Iranian Polymer Journal (English Edition). 2015; 24 (8):697-704. DOI: 10.1007/s13726-015-0361-0 - 149.
Liu K et al. Polyaniline Nanofiber Wrapped Fabric for High Performance Flexible Pressure Sensors. Polymers (Basel). 2019; 11 (1120):1-11. DOI: 10.3390/polym11071120 - 150.
Xu R et al. A flexible, conductive and simple pressure sensor prepared by electroless silver plated polyester fabric. Colloids and Surfaces A: Physicochemical and Engineering Aspects. 2019; 578 (February):123554. DOI: 10.1016/j.colsurfa.2019.04.096 - 151.
Ma Z, Wang W, Yu D. Assembled wearable mechanical sensor prepared based on cotton fabric. Journal of Materials Science. 2020; 55 (2):796-805. DOI: 10.1007/s10853-019-04035-0 - 152.
Celozzi S, Araneo R, Lovat G. Electromagnetic Shielding. Hoboken, New Jersey: John Wiley & Sons, Inc.; 2008. DOI: 10.1002/9780470268483 - 153.
Zubair K et al. Study of mechanical, electrical and EMI shielding properties of polymer-based nanocomposites incorporating polyaniline coated graphene nanoparticles. Nano Express. 2021; 2 (1):1-14. DOI: 10.1088/2632-959X/abe843 - 154.
Kim SH, Seong JH, Oh KW. Effect of dopant mixture on the conductivity and thermal stability of polyaniline/nomex conductive fabric. Journal of Applied Polymer Science. 2002; 83 (10):2245-2254. DOI: 10.1002/app.10211 - 155.
Onar N, Akşit AC, Ebeoglugil MF, Birlik I, Celik E, Ozdemir I. Structural, electrical, and electromagnetic properties of cotton fabrics coated with polyaniline and polypyrrole. Journal of Applied Polymer Science. 2009; 114 (4):2003-2010 - 156.
Zhou Z, Sun J. Fabrication and characterization of polyaniline on cotton fabric via two-step in-situ polymerization. Journal of Physics Conference Series. 2021; 1790 (1):012070. DOI: 10.1088/1742-6596/1790/1/012070 - 157.
Muthukumar N, Thilagavathi G. Development and characterization of electrically conductive polyaniline coated fabrics. Indian Journal of Chemical Technology. 2012; 19 (6):434-441 - 158.
Muthukumar N, Thilagavathi G, Kannaian T. Polyaniline-coated nylon lycra fabrics for strain sensor and electromagnetic interference shielding applications. High Performance Polymers. 2015; 27 (1):105-111. DOI: 10.1177/0954008314540313 - 159.
Dhawan SK, Singh N, Venkatachalam S. Shielding effectiveness of conducting polyaniline coated fabrics at 101 GHz. Synthetic Metals. 2001; 125 (3):389-393. DOI: 10.1016/S0379-6779(01)00478-7 - 160.
Joseph N, Varghese J, Sebastian MT. In situ polymerized polyaniline nanofiber-based functional cotton and nylon fabrics as millimeter-wave absorbers. Polymer Journal. 2017; 49 (4):391-399. DOI: 10.1038/pj.2016.121 - 161.
Pan T, Zhang Y, Wang C, Gao H, Wen B, Yao B. Mulberry-like polyaniline-based flexible composite fabrics with effective electromagnetic shielding capability. Composite Science Technology. 2020; 188 (December 2019):107991. DOI: 10.1016/j.compscitech.2020.107991 - 162.
Gulati R, Sharma S, Sharma RK. Antimicrobial textile: Recent developments and functional perspective. Polymer Bulletin. 2022; 79 (8):5747-5771. DOI: 10.1007/s00289-021-03826-3 - 163.
Maruthapandi M, Saravanan A, Luong JHT, Gedanken A. Antimicrobial properties of the polyaniline composites against pseudomonas aeruginosa and klebsiella pneumoniae. Journal of Functional Biomaterials. 2020; 11 (3):59. DOI: 10.3390/jfb11030059 - 164.
Bhandari V, Jose S, Badanayak P, Sankaran A, Anandan V. Antimicrobial finishing of metals, metal oxides, and metal composites on textiles: A systematic review. Industrial and Engineering Chemistry Research. 2022; 61 :86-101. DOI: 10.1021/acs.iecr.1c04203 - 165.
Unango FJ, Ramasamy KM. A review on the investigation of biologically active natural compounds on cotton fabrics as an antibacterial textile finishing. International Research Journal of Science and Technology. 2019; 1 (1):49-55 - 166.
Tan L et al. A review of antimicrobial fabric containing nanostructures metal-based compound. Journal of Vinyl and Additive Technology. 2019; 25 :E3-E27. DOI: 10.1002/vnl.21606 - 167.
Qian J et al. Highly stable, antiviral, antibacterial cotton textiles via molecular engineering. Nature Nanotechnology. 2023; 18 (2):168-176. DOI: 10.1038/s41565-022-01278-y - 168.
Robertson J, Gizdavic-Nikolaidis M, Nieuwoudt MK, Swift S. The antimicrobial action of polyaniline involves production of oxidative stress while functionalisation of polyaniline introduces additional mechanisms. PeerJ. 2018; 6 :1-36. DOI: 10.7717/peerj.5135 - 169.
Kucekova Z, Kasparkova V, Humpolicek P, Sevcikova P, Stejskal J. Antibacterial properties of polyaniline-silver films. Chemical Papers. 2013; 67 (8):1103-1108. DOI: 10.2478/s11696-013-0385-x - 170.
Seshadri DT, Bhat N. Use of polyaniline as an antimicrobial agent in textiles. Indian Journal of Fibre & Textile Research. 2005; 30 (2):204-206 - 171.
Meganathan P, Subbaiah S, Selvaraj LM, Subramanian V, Pitchaimuthu S, Srinivasan N. Photocatalytic self-cleaning and antibacterial activity of cotton fabric coated with polyaniline/carbon nitride composite for smart textile application. Phosphorus, Sulfur and Silicon and the Related Elements. 2022; 197 (3):244-253. DOI: 10.1080/10426507.2021.2012779