Abstract
Lewis acids have played a pivotal role in organic synthesis. The inherent Lewis acidity of semimetal or metal ions, which stems from possession of empty orbitals or electron-accepting ability/electron pair affinity, has granted them the ability to catalyze various and numerous organic functional group transformations and methods for carbon-carbon (C-C) bond formation reactions. Lewis acids have developed from conventional catalysts to modern green ones that have enabled a wide range of chemical transformations and chemical processes of applications in various fields, such as pharmaceutical and petroleum/petrochemical industries. The chapter begins with a definition and description of Lewis acids and their significance in chemistry as an electronic science. The chapter then covers the uses and applications of Lewis acids as homogeneous catalysts in conventional organic reactions that employ and/or are Lewis-acids catalysts. Coverage continues on the importance of developments and inception of green and environmentally benign Lewis acids that can help preserve the environment, in a manifestation of green chemistry principles. Illustrations of the importance and applications of green Lewis acids in Lewis acid-catalyzed organic reactions and C-C bond formation reactions are covered. The chapter aims at introducing and stimulating the reader to Lewis acids, their description, and significance in synthetic organic chemistry in particular and in chemistry as a whole.
Keywords
- Lewis acids
- empty orbitals
- Lewis-acid catalysis
- homogenous catalysts
- green Lewis acids
1. Introduction
According to Gilbert Lewis, a Lewis acid is a substance (atom, ion, or molecule) that contains or possesses an empty/vacant or unfilled atomic or molecular orbital that is capable of accepting or accommodating an electron pair [1, 2, 3, 4, 5, 6, 7, 8]. This implies that substances with an incomplete or unsatisfied octet act as Lewis acids. An electron pair donor, a Lewis base, performs the donation of the electron pair from its highest occupied molecular orbital (HOMO) to the lowest occupied molecular orbital (LUMO) of the Lewis acid (Figure 1). Thus, in simple terms, a Lewis acid is an electron pair acceptor. The process of accepting an electron pair by a Lewis acid from a Lewis base results in the formation of a Lewis complex or adduct with a new coordinate covalent bond/dative bond or σ bond. Lewis acidity is closely linked and intertwined with electrophilicity. Thus, due to their electron deficiency, electrophiles with positive or partially positive characters as a result of electronic effects (inductive and electronic) manifest themselves as electron pair acceptors. Hence, Lewis acidity parallels electrophilicity in a similar manner that Lewis basicity parallels nucleophilicity.
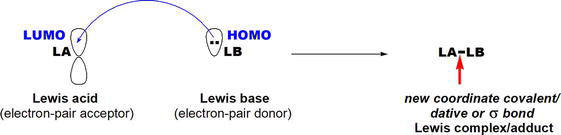
Figure 1.
The general reaction between a Lewis acid and a Lewis base.
Typical general examples of Lewis acids are based on B or boranes (BR3) [6], such as BH3 and BF3 and aluminum compounds, AlR3, such as AlCl3. Other examples include alkali (M+) metal ions, such as K+ and alkaline earth metal ions (M2+), such as Ca2+ and transition metal ions, such as Mn2+. Other examples of Lewis acids include the proton, H+, carbocations, R3C+ [8], and electron-deficient π-systems. The vast majority of Lewis acids are metal-based, such as those based on Al, Ti, Mg, and Mn. Some are metalloid/semimetal-based, such as those based on B [6] and Si [7]. A typical example of a Lewis acid-Lewis base reaction is the reaction between BF3 (Lewis acid) and NH3 (Lewis base) (Figure 2).
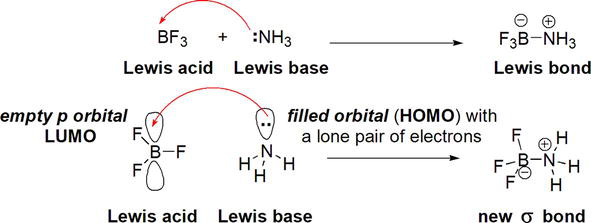
Figure 2.
Reaction between BF3 (Lewis acid) and NH3 (Lewis base).
Conventional Lewis acids have long been used as catalysts and played significant roles in various chemical transformations, particularly organic synthetic reactions. Notably, in oxidation and reduction reactions, electrophilic aromatic substitution reactions exemplified by Friedel-Crafts alkylation and acylation, and halogenation reactions of benzene. Conventional Lewis acids have catalyzed other numerous organic reactions typified by the Diels-Alder reaction, Alder-ene reaction, aldol reaction, reactions of silyl enol ethers (direct (1,2-) addition, and conjugate (1,4-) addition) and acylation of ketones.
2. Conventional uses of Lewis acids in organic synthesis
Lewis acids have been used and applied in numerous organic transformations. They have been in various functional group transformations and C-C/C-X bond formation reactions [9, 10, 11]. Below are selected representative reactions that are only intended to highlight the importance of Lewis acids in organic synthesis but by no means a comprehensive review of all organic transformations catalyzed by Lewis acids. For additional details, the reader is advised to consult the original literature.
2.1 Oxidation reactions
Primary alcohols and secondary alcohols can be oxidized to the corresponding aldehydes and ketones, respectively, using TPAP/NMO oxidation method (TPAP: tetrapropylammonium perruthenate, NMO:
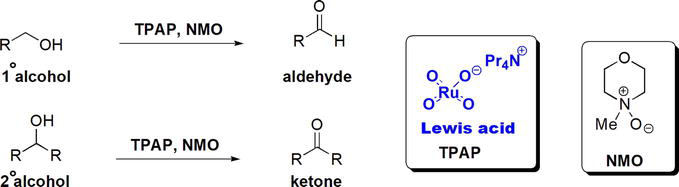
Figure 3.
Oxidation of alcohols using Lewis acidic TPAP/NMO.
The perruthenate ion acts as a Lewis acid on its Ru center (Figure 4). The reaction is initiated by the reaction of the Lewis basic alcohol and the Lewis acidic Ru on the perruthenate ion to give an intermediate that leads to the product aldehyde. The resultant Lewis acidic Ru (RuO3H) species then reacts with the O-Lewis basic NMO to regenerate the Lewis acidic perruthenate catalyst.
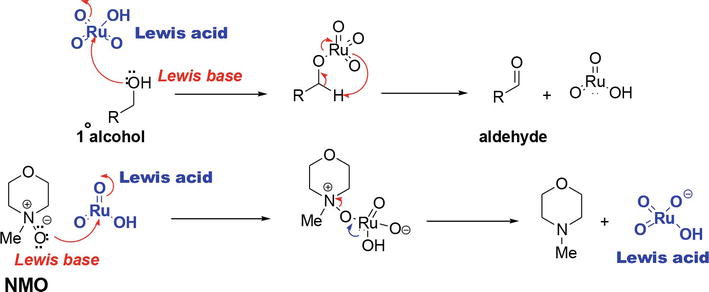
Figure 4.
Mechanism of the TPAP/NMO oxidation of alcohols.
Allylic and benzylic alcohols can be selectively oxidized to the corresponding aldehydes using activated MnO2 (Figure 5) [13, 14].
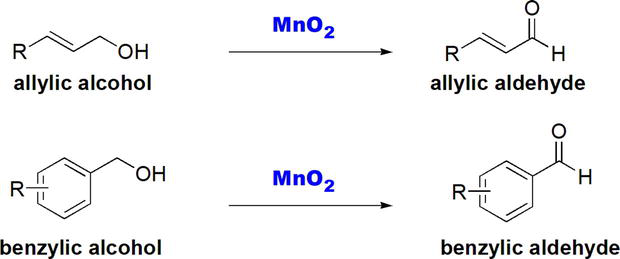
Figure 5.
Oxidation of allylic and benzylic alcohols with MnO2.
In the reaction, MnO2 functions as a Lewis acid that accepts an electron pair from the Lewis basic O of the alcohol (Figure 6).

Figure 6.
The reaction between the Lewis acidic MnO2 with allylic alcohol.
It has been reported that oxidation of alcohols to the corresponding ketones with potassium permanganate, KMnO4, can be accelerated using Lewis acids, such as BF3, Sc(OT
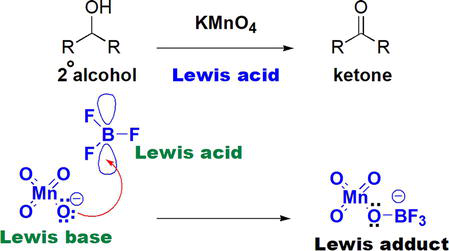
Figure 7.
Acceleration of alcohol KMnO4 oxidation by Lewis acids.
Oxidation of sulfides to the corresponding ketones by KMnO4 could be enhanced using the Lewis acidic FeCl3 (Figure 8) [16]. The reaction is initiated by a Lewis complex that is formed upon the reaction of the Lewis basic permanganate, MnO4−, and the Lewis acidic FeCl3 (Figure 8).

Figure 8.
Acceleration of sulfide KMnO4 oxidation by Lewis acidic FeCl3.
2.2 Reduction reactions
Esters can be reduced to the corresponding aldehydes using DIBAL-H (diisobutylaluminum hydride) at very low temperatures (̶ 78°C) [17]. DIBAL-H acts as a Lewis acid, thus accepting an electron pair from the Lewis basic ester (Figure 9). The conversion of the ester into the corresponding aldehyde lies in the formation of the Lewis complex that leads to a tetrahedral intermediate that leads to the aldehyde upon aqueous workup.
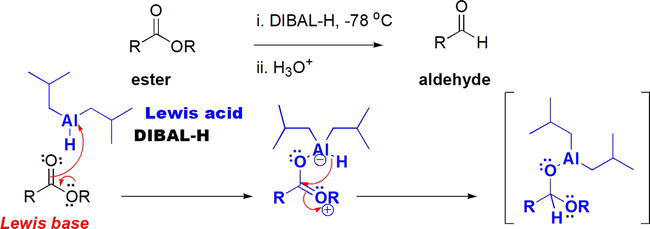
Figure 9.
Reduction of esters with Lewis acidic DIBAL-H at very low temperature.
Lewis acids have been used as reducing agents in various functional group transformations. Based on the electron deficiency of Lewis acids, which stems from the presence of empty orbitals, they react with electron-rich carboxylic acids and carboxylic acid derivatives, such as amides. In this regard, borane, BH3, is found to be an excellent reducing agent for amides [18] and carboxylic acids [19] to primary amines (Figure 10) and primary alcohols, respectively. Borane, BH3, is a mild reducing agent that is used instead of the more reactive LiAlH4 that requires special handling procedures. The gaseous BH3 is conveniently used as a liquid complex with diethyl ether (BH3.Et2O), tetrahydrofuran (BH3.THF), or dimethyl sulfide (BH3.DMS). The utility of BH3 as an amide-reducing agent is extended to its chemoselective reduction ability of amides in the presence of less electron-rich carboxylic acid derivatives such as esters.
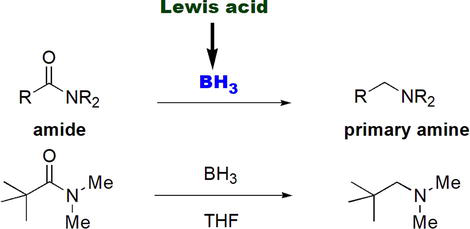
Figure 10.
Reduction of amides using borane, BH3.
Mechanistically, amides utilize the

Figure 11.
The mechanism for the reduction of amides using borane, BH3.
Similarly, carboxylic acids can conveniently be reduced with borane to give the corresponding primary alcohols (Figure 12). Borane has proven to be a highly efficient chemoselective reducing agent for carboxylic acids in the presence of less electron-rich carbonyl groups such as esters (Figure 12).
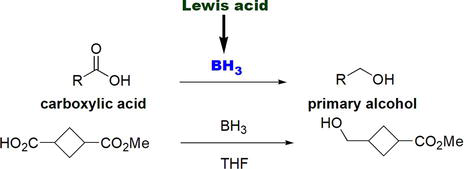
Figure 12.
Reduction of carboxylic acids using borane, BH3.
Sodium borohydride, NaBH4, is known as a mild reducing agent for aldehydes and ketones. If an α,β-unsaturated carbonyl compound, such as the α,β-unsaturated ketone shown (Figure 13), is treated with NaBH4, reduction of the both carbonyl group and the double bond takes place. Thus, the reaction is not regioselective/chemoselective. Chemoselective reduction of the carbonyl group in α,β-unsaturated ketone, while keeping the double bind intact, can be nicely achieved by adding the Lewis acidic cerium chloride, CeCl3 (Figure 13). The NaBH4-CeCl3 reduction method is known as Luche reduction [20].
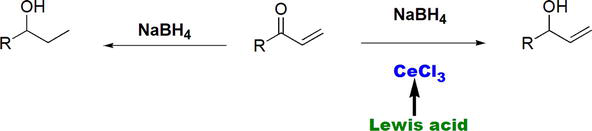
Figure 13.
Chemoselective reduction of α,β-unsaturated carbonyl compounds using NaBH4-CeCl3 reduction (Luche reduction).
The role of the Lewis acidic CeCl3 lies in its reaction with Lewis basic O of the carbonyl group. That is by donation of an electron pair from O to an empty orbital in Ce (Figure 14). The Lewis complex produced facilitates subsequent and chemoselective reduction of the ketone by NaBH4/CeCl3 in the presence of the C=C bond.
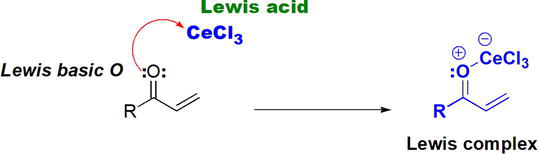
Figure 14.
The role of CeCl3 in NaBH4-CeCl3 reduction (Luche reduction).
2.3 Electrophilic aromatic substitution: Friedel-crafts alkylation and acylation
Traditionally, Lewis acids have been used as catalysts in electrophilic aromatic substitution reactions of benzenes as typified by Friedel-Crafts alkylation and acylation reactions. The Friedel-Crafts alkylation employs benzene as a starting reactant that is reacted with an alkyl halide in the presence of AlX3 as a catalyst (Figure 15) [21].
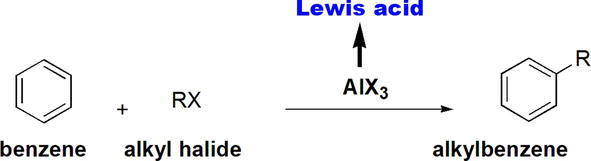
Figure 15.
Friedel-crafts alkylation of benzene.
Due to the inherent stability of benzene, the Lewis acid catalyst is essential for the reaction. That is, no reaction would take place in the absence of a Lewis acid catalyst. Benzene needs a highly electrophilic species to react with in order for the electrophilic aromatic substitution to take place. Although alkyl halides are electrophilic by inductive effect, they are not sufficiently electrophilic for benzene to lose aromaticity and react with them. This will require a high-energy barrier to overcome. The role of the AlX3 Lewis acid catalyst lies in its reaction with the Lewis basic alkyl halide. The alkyl halide donates one of the electron pairs on the halogen to the empty p orbital on Al in AlX3. That is employing a HOMO from X in RX to the LUMO on Al in AlX3. This results in the formation of the Lewis adduct, where a new σ bond between X and Al has formed. This Lewis adduct is a highly electrophilic species that is sufficiently electrophilic for the stable nucleophilic benzene (Figure 16).
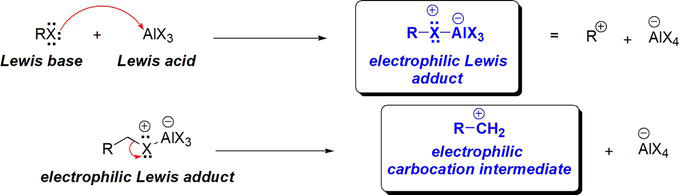
Figure 16.
Role of Lewis acidic AlCl3 in Friedel-crafts alkylation of benzene.
The resultant Lewis adduct imparts a positive/electrophilic character on C bonded to X in the adduct. From a mechanistic point of view, this intermediate is susceptible to benzene nucleophilic attack. Alternatively, the electrophilic Lewis adduct can also be envisioned to undergo dissociation to an electrophilic carbocation intermediate setting stage for nucleophilic attack by benzene (Figure 16). The figure below shows examples of Friedel-Crafts alkylation of benzene (Figure 17).
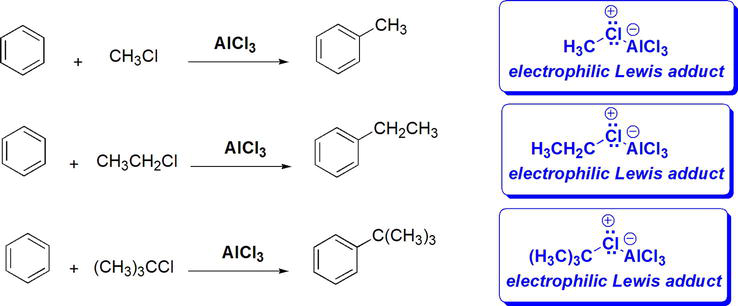
Figure 17.
Examples of Friedel-crafts alkylation of benzene.
Friedel-Crafts acylation of benzene is the reaction of benzene with an acid halide (or anhydride) in the presence of a Lewis acidic AlX3 (Figure 18) [21].
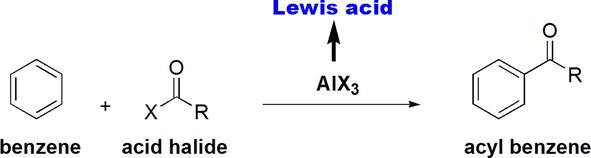
Figure 18.
Friedel-crafts acylation of benzene.
As in the Friedel-Crafts alkylation reaction, the Lewis acid catalyst is essential for the Friedel-Crafts acylation reaction. The role of the AlX3 Lewis acid catalyst lies in its reaction with the Lewis basic acid halide. The acid halide acts as a Lewis base employing one of the electron pairs on the halogen. Thus, an electron pair is donated from the Lewis basic X to the empty p orbital on Al in AlX3. This results in the formation of the Lewis adduct, where a new σ bond between X and Al has formed. This Lewis adduct is a highly electrophilic species that is sufficiently electrophilic rendering its susceptibility to nucleophilic attack by benzene (Figure 19).
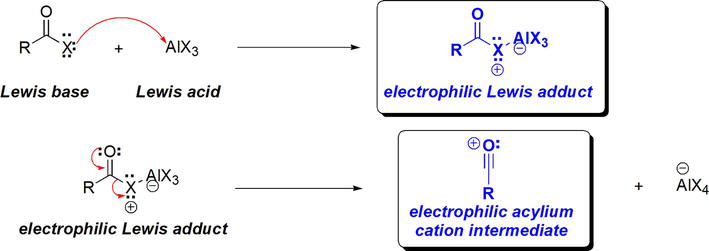
Figure 19.
Role of Lewis acidic AlCl3 in Friedel-crafts acylation of benzene.
The electrophilic Lewis adduct formed can be envisioned to undergo dissociation to a highly electrophilic acylium cation intermediate. Thus, the stage is set for a facile Friedel-Crafts acylation reaction (Figure 19). The figure below shows examples of Friedel-Crafts acylation of benzene (Figure 20).

Figure 20.
Examples of Friedel-crafts acylation of benzene.
2.4 Electrophilic aromatic substitution: Halogenation
Halogenation of benzene using a halogen in the presence of FeX3 is an example of electrophilic aromatic substitution reactions (Figure 21). In the reaction, FeX3 acts as a Lewis acid, which is essential for the reaction [22].
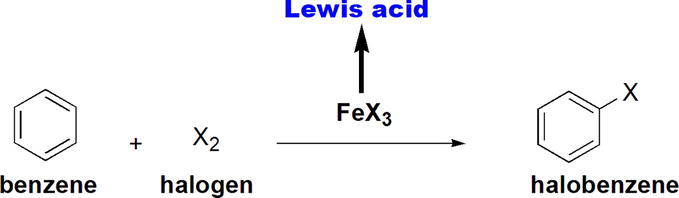
Figure 21.
Halogenation of benzene using X2 in the presence of FeX3.
The Lewis basic halogen, X2 donates an electron pair from X to an empty orbital on Fe in FeX3 to form an electrophilic Lewis adduct (Figure 22). This electrophilic intermediate is susceptible to nucleophilic attack by benzene at the terminal halogen (X) of the polarized X-X bond. This electrophilic Lewis adduct can be envisioned to undergo dissociation of the polarized X-X bond to give X+ as an equivalent electrophilic intermediate (Figure 22). Thus, the stage is set for a benzene nucleophilic attack.
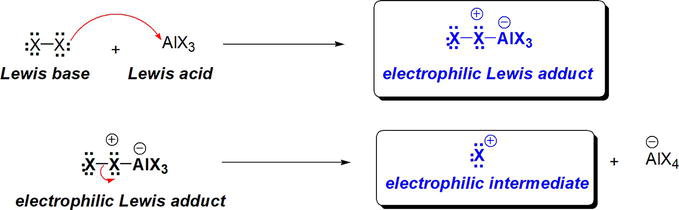
Figure 22.
Role of Lewis acidic FeX3 in Friedel-crafts acylation of benzene.
The Figure below shows examples of the halogenation of benzene (Figure 23).

Figure 23.
Examples of halogenation of benzene using X2 in the presence of FeX3.
2.5 Hydroboration-oxidation
Hydroboration-oxidation is an electrophilic addition of borane, BH3, to alkenes or alkynes. In the case of hydroboration of alkenes, the product is alcohol that ends at the less sterically hindered or more accessible C of C=C bond of the alkene (Figure 24) [23]. This method is a complementary method to the oxymercuration of alkenes that leads to the more substituted alcohol. Unlike oxymercuration, which follows Markovnikov’s rule, hydroboration-oxidation is an anti-Markovnikov’s alcohol formation. BH3 acts as a Lewis acid/electrophile that utilizes its empty p orbital to accept an electron pair from the nucleophilic double bond in an alkene. The hydroboration addition takes place in a concerted manner, thus governed by steric control. As a result, the BH2 group of the electrophilic BH3 ends up at the less sterically hindered (more accessible) C of the C=C bond, while H bonds to the more sterically hindered C (less accessible).

Figure 24.
Hydroboration-oxidation of alkenes.
2.6 Diels-Alder reaction
The Diels-Alder reaction is an electrocyclic [4 + 2] cycloaddition reaction between a diene and a dienophile [24, 25]. It is a robust and well-established C-C formation method. Typically, the diene is bonded with an electron-donating group (EDG), such as alkoxy (OR) or amino groups (NR2), and thus electron-rich. The dienophile is typically electron-poor, because of attachment with an electron-withdrawing group (EWG), such as an ester (CO2R), cyano (CN), or nitro (NO2) groups (Figure 25). The regioselectivity is governed by proper interactions between the HOMO of the diene and the LUMO of the dienophile (Figure 26).
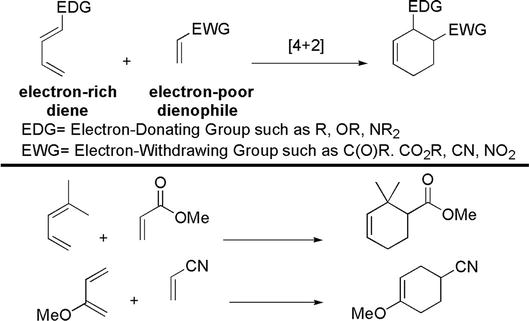
Figure 25.
Diels-Alder reaction.
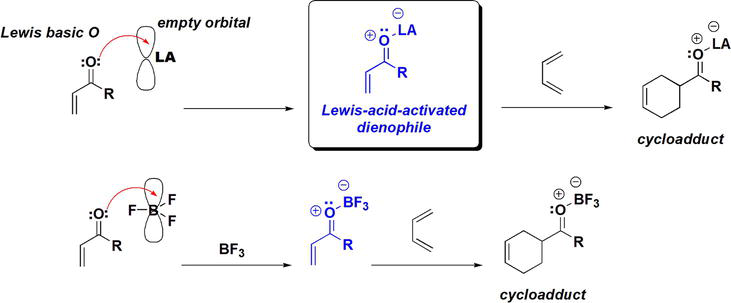
Figure 26.
Role of Lewis acids in activation of dienophiles as reaction partners in Diels-Alder reactions.
Diels-Alder reactions that do not meet the proper electron-demand/match involving an electron-rich diene and electron-poor dienophile may require Lewis acid catalysis. The role of Lewis acids lies in their activation of the Lewis basic dienophile by binding with it. Formation of the Lewis adduct results in lowering the LUMO of the dienophile (Figure 26). The result is a smaller energy gap between the HOMO of the diene and the LUMO of the dienophile, setting the stage for stronger orbital interactions and thus enhancement of reaction rates. Lewis acids, such as AlCl3, BF3, ZnCl2, and TiCl4, have been found to be efficient catalysts for the Diels-Alder reaction.
2.7 Alder-ene reaction
The Alder-ene reaction is an electrocyclic cycloaddition reaction between an ene and an enophile (Figure 27) [26, 27].
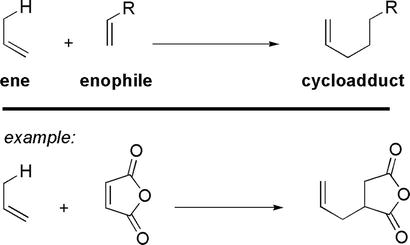
Figure 27.
The Alder-ene reaction.
The role of Lewis acids in the Alder-ene reaction lies in their activation of the enophile by binding with it thus lowering the LUMO of the enophile (Figure 28). As a result, the energy gap between the HOMO of the ene and the LUMO of the enophile is lowered. Therefore, the resultant stronger interactions facilitate the ene reaction.
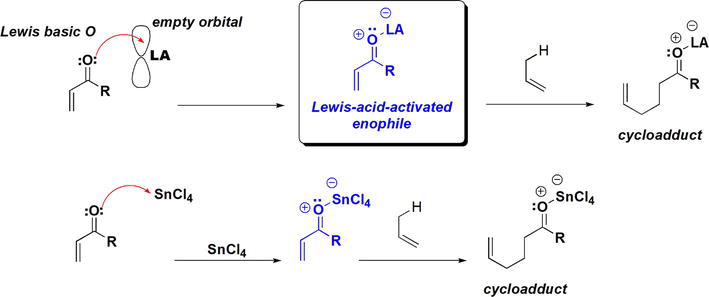
Figure 28.
Role of Lewis acids in activation of enophiles in the Alder-ene reaction.
2.8 Mukaiyama aldol reaction
The aldol reaction, which is a reaction between an enolate of a carbonyl compound and an aldehyde or ketone to form β-hydroxy carbonyl compound or α,β-unsaturated carbonyl compound. The Mukaiyama aldol reaction is a variant of the aldol reaction that employs a silyl enol ether, an enolate equivalent, as a nucleophile, an aldehyde or a ketone as an electrophile in the presence of TiCl4 as a Lewis acid catalyst (Figure 29) [28].
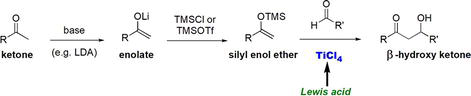
Figure 29.
The Mukaiyama aldol reaction employs TiCl4 as a Lewis acid.
The role of the TiCl4 Lewis acid catalyst lies in its coordination with the electrophilic aldehyde or ketone. The Lewis basic O of the carbonyl group employs one of its lone pairs for donation to an empty orbital on the Lewis acidic Ti in TiCl4. The result is the formation of a Lewis adduct that is an activated aldehyde with enhanced electrophilicity. The highly electrophilic aldehyde-TiCl3 Lewis complex sets the stage for subsequent nucleophilic attack by the silyl enol ether (Figure 30). The reaction continues to ultimately give the β-hydroxy carbonyl compound.
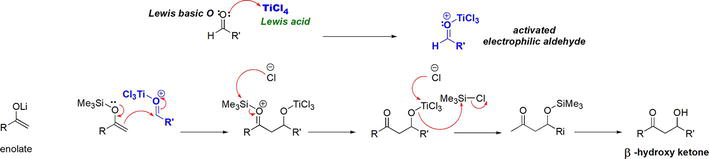
Figure 30.
The role of the Lewis acidic TiCl4 in the Mukaiyama aldol reaction.
2.9 C-F bond functionalization
The synthetic utility of Lewis acids has been extended to C-F bond functionalization reactions [29]. Lewis acids based on B, Si, and Al have affected the hydrodefluorination of alkyl fluorides, which is the transformation of C-F bond into C-H bond (Figure 31). Lewis acids based on B, Al, and Si (Figure 30) have achieved the ultimate functionalization of C-F bonds. Functionalization of C-F bonds has been achieved due to the high Lewis acidity of B, Al, and Si and to the high fluoride affinity, especially in the case of Si.
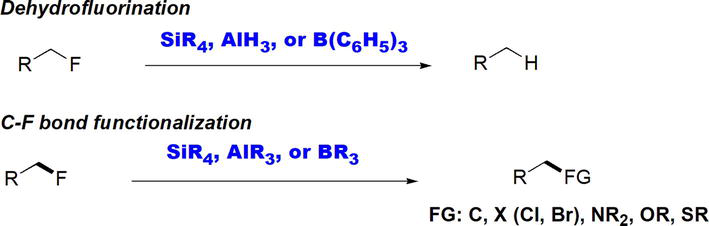
Figure 31.
Lewis acid-assisted C-F bond functionalization.
3. Green Lewis acids
3.1 Uses in various organic reactions
Typical Lewis acids, such as BF3 and AlCl3, are highly reactive with water. Thus, they are non-recoverable and end up as waste at the end of reactions. Catalysis is one of the 12 principles of green chemistry and this is due to the minimization of waste at the end of the reaction. Prevention of waste is another principle of the 12 principles of green chemistry. Therefore, it is a viable strategy to seek and develop green Lewis acid alternatives to the highly water-sensitive Lewis acids, such as BF3 and AlCl3 [30]. From a green chemistry point of view, the alternative should still function as a catalyst and it should not be water-sensitive. The idea is to minimize waste that is obtained at the end of reactions that employ BF3 and AlCl3. Lanthanide triflates (trifluoromethanesulfonate) are water-soluble and recyclable. The triflate group is a strongly electron-withdrawing group that imparts or enhances the Lewis acidity of the lanthanide. Thus, they are considered green Lewis acids. Examples of lanthanide triflates are Yb(OTf)3, Sc(OT
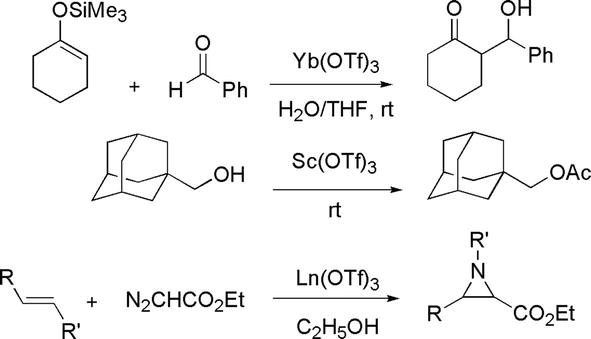
Figure 32.
Lanthanide triflates as Lewis acids in organic reactions.
Developments of green alternatives to the classical stoichiometric oxidants, such as CrO3 and MnO2, that employ heavy metals producing metal waste, thus posing environmental problems, are sought. Ideal oxidants should be stable, active, and selective and ideally based on green oxidants, such as air or dilute H2O2. An example of green Lewis acidic oxidant is TS-1 (titanium silicate) (Figure 33) [32]. TS-1 is especially useful for oxidation reactions using H2O2 where the only by-product is water. Examples of its use include its catalytic conversion of cyclohexanone into cyclohexanone oxime, which is elaborated into caprolactam, a nylon 6 precursor (Figure 33).
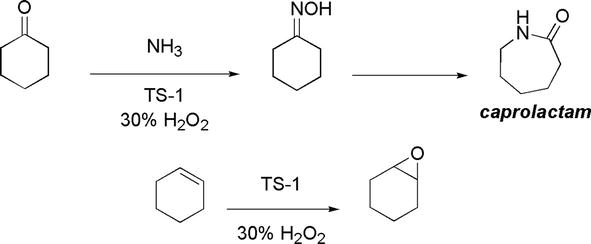
Figure 33.
Use of TS-1 in organic reactions.
A green Lewis acid-based zeolite, Sn-beta, was developed. Sn-beta [33] has been found to catalyze various oxidation methods/processes such as Baeyer-Villiger oxidation reaction of ketones to produce the corresponding esters. Typical oxidations of alkylbenzene or aromatic compounds involve HNO3/H2SO4 oxidation method (Figure 34). A green alternative to the harsh Lowry-Bronsted acidic method is using mild Lewis acid. An oxidation method that employs V2O5 and TiO2 is found to oxidize 3-methyl pyridine into nicotinic acid [34].

Figure 34.
Use of Lewis acids as green oxidant toward nicotinic acid.
TPAP (tetrapropylammonium perruthenate),
3.2 Aqueous Diels-Alder reaction
The Diels-Alder reaction is one of the great C-C bond formation reactions. Its great utility in organic synthesis has been extended to its ability to take place in the presence of water in organic solvents or in water as the sole solvent [36, 37, 38]. Rates of Diels-Alder reactions have been tremendously accelerated in water/aqueous media. The enhancement of reaction rates is attributed to the association of water molecules with each other forcing organic molecules to come in proximity with each other and thus close contact commencing reactions. Lewis acid-catalyzed Diels-Alder reactions that have been carried out in water/in the presence of water have demonstrated the hydrophobic effect. Examples are shown below (Figure 35). Lewis acid-catalyzed aqueous Diels-Alder reactions manifest themselves as green organic reactions by fulfilling some great green chemistry principles, such as catalysis, prevention of waste, and use of environmentally benign solvents.
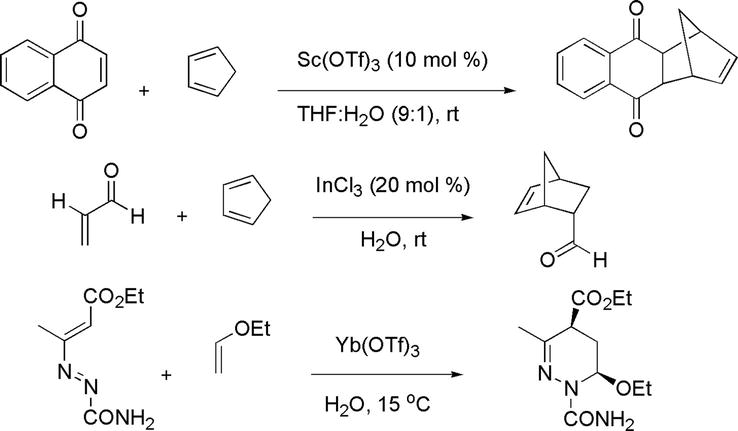
Figure 35.
Examples of Lewis acid-catalyzed Diels-Alder reactions in water.
3.3 Aqueous aldol reaction
The aldol reaction is one of the great C-C bond formation reactions in organic synthesis. Their ability to take place in the presence of water has extended their utility/versatility in organic synthesis. Lewis acid-catalyzed aqueous aldol reactions have been possible employing silyl enol ether as masked nucleophilic enolates and a wide range of metal chloride, perchlorate of triflate (Figure 36) [31, 38].
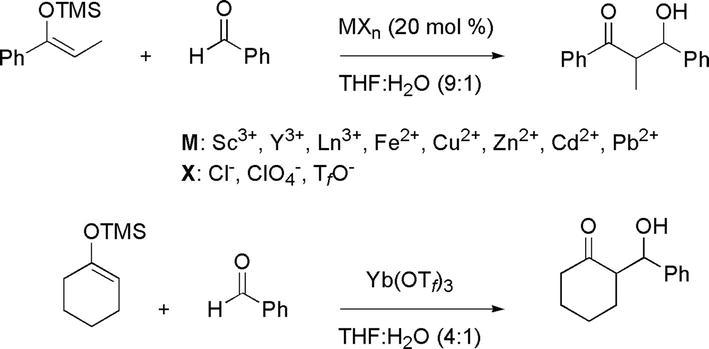
Figure 36.
Examples of Lewis acid-catalyzed aldol reactions in water.
3.4 As ionic liquids
Ionic liquids are produced from large, non-coordinating asymmetric ions with one of them being organic. Usually, the organic ion is a bulky heterocyclic cation that interacts with a small inorganic anion. Due to difference in size, the ion packing in ionic liquids is poor, which makes them liquids at room temperature. Examples of ionic liquid include [(emim)AlCl4], [(bmim)BF4], and [(bmim)PF6] (Figure 37) [39].

Figure 37.
Examples of ionic liquids.
The positive/electrophilic character of the bulky organic ion imparts Lewis acidity to ionic liquids. Thus, they are used as catalysts. Ionic liquids Lewis acidic catalysts that are not miscible with organic solvents are separable from reaction mixtures, thus recoverable and recyclable/reusable. Therefore, catalytic Lewis acidic ionic liquids are green Lewis acids. Reactions involving ionic liquids may result in simpler workup procedures, especially if the organic product is immiscible with the ionic liquid. This allows easy and simple product separation and purification. [(emim)AlCl4] has been used as a catalyst in oligomerization/polymerization reactions that occurred with a high reaction rate and conversions. The Lewis acidity of ionic liquids can be enhanced by increasing the amount of the Lewis acid, the counter anion, in the formation process. Ionic liquids have been immobilized for uses and applications in alkylation reactions of aromatic compounds, such as benzene, toluene, and naphthalene with olefins [39].
3.5 Oil refining
Cracking of heavy oil fractions is required for the efficient production of gasoline. Original catalytic cracking methods were carried out thermally employing AlCl3 as a Lewis acid. Such processes were considered inefficient due to their high-energy intensive feature and also due to the use of wasteful, corrosive, and environmentally non-friendly Lewis acid. Catalytic cracking currently and commonly employs zeolites such as zeolite Y. Zeolites are made of three-dimensional frameworks of crystalline hydrated aluminosilicates consisting of TO4 (T: tetrahedral, Si or Al). Zeolites are hydrated, which imparts significant Brønsted acidity (Figure 38) [40].
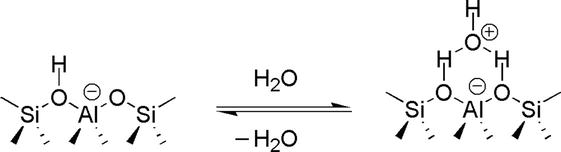
Figure 38.
The structure of zeolites.
The exact nature of zeolite is determined by important parameters, such as reaction conditions and
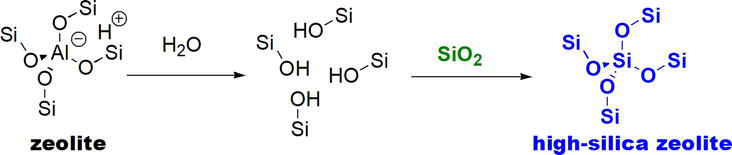
Figure 39.
Dealumination of zeolites and formation of high silica zeolites.
High silica zeolites result in reactions with high yields and better selectivity. Green development is the
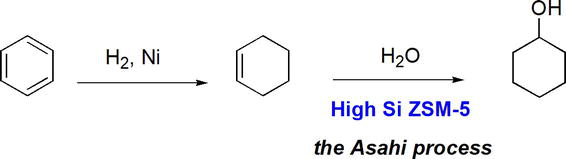
Figure 40.
Use of high silica zeolite.
4. Concluding remarks
Due to their electron-pair affinity, Lewis acid catalysts have catalyzed various and numerous organic reactions, and C-C bond formation methods and processes. Their utility has been extended to their compatibility with water as a reaction medium. The catalytic feature of Lewis acids and the ability to use them in aqueous media make them environmentally benign and thus green catalysts. Applications of Lewis acids have been demonstrated by their ability to catalyze various organic transformations/reactions and in the ultimate and constantly sought reaction medium, water. The power of Lewis acids has affected the challenging C-F bond functionalization reactions. Lewis acids have seen applications in green processes/methods, such as ionic liquids, and in industrial processes, such as catalytic cracking in oil refining. It is envisioned that Lewis acids will have the potential to further contribute in the field of C-X bond functionalization reactions and other green transformations/processes.
References
- 1.
Lewis GN. Valence and the Structure of Atoms and Molecules. New York: The Chemical Catalog Co; 1923. DOI: 10.1002/jctb.5000430107 - 2.
Lewis GN. The atom and the molecule. Journal of the American Chemical Society. 1916; 38 :762-785. DOI: 10.1021/ja02261a002 - 3.
Pearson RG. Absolute electronegativity and absolute hardness of Lewis acids and bases. Journal of the American Chemical Society. 1985; 107 :7801-6806. DOI: 10.1021/JA00310A009 - 4.
Brown ID, Skowron A. Electronegativity and Lewis acid strength. Journal of the American Chemical Society. 1990; 112 :3401-3403. DOI: 10.1021/ja00165a023 - 5.
Kobayashi S, Busujima T, Nagayama S. A novel classification of Lewis acids based on activity and selectivity. Chemistry - A European Journal. 2000; 6 :3491-3494. DOI: 10.1002/1521-3765(20001002)6:19%3C3491::AID-CHEM3491%3E3.0.CO;2-P - 6.
Sivaev IB, Bregadze VI. Lewis acidity of boron compounds. Coordination Chemistry Reviews. 2014; 270–271 :75-88. DOI: 10.1016/j.ccr.2013.10.017 - 7.
Dilman AD, Ioffe SL. Carbon-carbon bond forming reactions mediated by silicon Lewis acids. Chemical Reviews. 2003; 103 :733-772. DOI: 10.1021/cr020003p - 8.
Naidu VR, Ni S, Franzén J. The carbocation: A forgotten Lewis acid catalyst. ChemCatChem. 2015; 7 :1896-1905. DOI: 10.1002/cctc.201500225 - 9.
Corma A, Hermenegildo G. Lewis acids as catalysts in oxidation reactions: From homogeneous to heterogeneous systems. Chemical Reviews. 2002; 102 :3837-3892. DOI: 10.1021/cr010333u - 10.
Yamamoto Y. From ó- to ð-electrophilic Lewis acids. Application to selective organic transformations. The Journal of Organic Chemistry. 2007; 72 :7817-7831. DOI: 10.1021/jo070579k - 11.
Suzuki K. Novel Lewis acid catalysis in organic synthesis. Pure and Applied Chemistry. 1994; 66 :1557-1564. DOI: 10.1351/pac199466071557 - 12.
Ley SB, Norman J, Griffith WP, Marsden SP. Tetrapropylammonium perruthenate, Pr4N+RuO4−, TPAP: A catalytic oxidant for organic synthesis. Synthesis. 1994; 7 :639-666. DOI: 10.1055/s-1994-25538 - 13.
Harfenist M, Bavley A, Lazier WA. The oxidation of allyl and benzyl alcohols to the aldehydes. The Journal of Organic Chemistry. 1954; 19 :1608-1616. DOI: 10.1021/jo01375a008 - 14.
Gritter RJ, Wallace TJ. The manganese dioxide oxidation of allylic alcohols. The Journal of Organic Chemistry. 1959; 24 :1051-1056. DOI: 10.1021/jo01090a006 - 15.
Du H, Lo P-K, Hu Z, Liang H, Lau K-C, Wang Y-N, et al. Lewis acid-activated oxidation of alcohols by permanganate. Chemical Communications. 2011; 47 :7143-7145. DOI: 10.1039/C1CC12024G - 16.
Lai S, Lee DG. Lewis acid assisted permanganate oxidations. Tetrahedron. 2002; 58 :9879-9887. DOI: 10.1016/S0040-4020(02)01227-9 - 17.
Zakharkin LI, Khorlina IM. Reduction of esters of carboxylic acids into aldehydes with diisobutylaluminium hydride. Tetrahedron Letters. 1962; 3 :619-620. DOI: 10.1016/S0040-4039(00)70918-X - 18.
Kornet MJ, Thio PA, Tan SI. Borane reduction of amido esters. The Journal of Organic Chemistry. 1968; 33 :3637-3639. DOI: 10.1021/jo01273a063 - 19.
Yoon NM, Cho BT. Rapid reduction of carboxylic acid salts with borane in tetrahydrofuran. Tetrahedron Letters. 1982; 23 :2475-2478. DOI: 10.1016/S0040-4039(00)87372-4 - 20.
Luche JL. Lanthanides in organic chemistry. 1. Selective 1,2 reductions of conjugated ketones. Journal of the American Chemical Society. 1978; 100 :2226-2227. DOI: 10.1021/ja00475a040 - 21.
Calloway NO. The Friedel-crafts syntheses. Chemical Reviews. 1935; 17 :327-392. DOI: 10.1021/cr60058a002 - 22.
de la Mare PBD. Electrophilic Halogenation Reaction Pathways Involving Attack by Electrophilic Halogens on Unsaturated Compounds. London: Cambridge University Press; 1976 - 23.
Brown HC, Kulkarni SV, Khanna VV, Patil VD, Racherla US. Organoboranes for synthesis. 14. Convenient procedures for the direct oxidation of organoboranes from terminal alkenes to carboxylic acids. The Journal of Organic Chemistry. 1992; 57 :6173-6177. DOI: 10.1021/jo00049a024 - 24.
Fringuelli F, Taticchi A. The Diels-Alder Reaction: Selected Practical Methods. John Wiley & Sons; 2001 - 25.
Vermeeren P, Tiezza MD, van Dongen M, Fernández I, Bickelhaupt FM, Hamlin TA. Lewis acid-catalyzed Diels-Alder reactions: Reactivity trends across the periodic table. Chemistry - A European Journal. 2021; 27 :10610-10620. DOI: 10.1002/chem.202100522 - 26.
Brummond KM, Loyer-Drew JA. 10.12 - C–C bond formation (part 1) by addition reactions: Alder-ene reaction. Comprehensive Organometallic Chemistry III. 2007; 10 :557-601. DOI: 10.1016/B0-08-045047-4/00134-5 - 27.
Tieknik EH, Vermeeren P, Bickelhaupt FM, Hamlin TA. How Lewis acids catalyze ene reactions. European Journal of Organic Chemistry. 2021:5275-5283. DOI: 10.1002/ejoc.202101107 - 28.
Matsuo J-I, Murakami M. The Mukaiyama aldol reaction: 40 years of continuous development. Angewandte Chemie, International Edition. 2013; 52 :9109-9118. DOI: 10.1002/anie.201303192 - 29.
Stahl T, Klare HFT, Oestreich M. Main-group Lewis acids for C−F bond activation. ACS Catalysis. 2013; 3 :1578-1587. DOI: 10.1021/cs4003244 - 30.
Kobayashi S, Manabe K. Green Lewis acid catalysis in organic synthesis. Pure and Applied Chemistry. 2000; 72 :1373-1380. DOI: 10.1351/pac200072071373 - 31.
Kobayashi S, Ogawa C. New entries to water-compatible Lewis acids. Chemistry - A European Journal. 2006; 12 :5954-5960. DOI: 10.1002/chem.200600385 - 32.
Xiong G, Hu D, Guo Z, Meng Q, Liu L. An efficient titanium silicalite-1 catalyst for propylene epoxidation synthesized by a combination of aerosol-assisted hydrothermal synthesis and recrystallization. Microporous Mesoporous Materials. 2018; 268 :93-99. DOI: 10.1016/j.micromeso.2018.04.015 - 33.
Zhu Z, Xu H, Jiang J, Guan Y, Wu P. Sn-Beta zeolite hydrothermally synthesized via interzeolite transformation as efficient Lewis acid catalyst. Journal of Catalysis. 2017;352 :1-12. DOI: 10.1016/j.jcat.2017.04.031 - 34.
Vorobyev P, Mikhailovskaya T, Yugay O, Saurambaeva L, Serebryanskaya A, Chukhno N, et al. Optimization of vanadium oxide catalyst for the oxidation of 3-methylpyridine into nicotinic acid. Journal of the Serbian Chemical Society. 2017; 82 :791-801. DOI: 10.2298/JSC161220023Z - 35.
Ciriminna R, Carraro M, Campestrini S, Pagliaro M. Sol-gel entrapped TPAP: An off-the-shelf catalyst set for the clean oxidation of alcohols. Current Organic Chemistry. 2008; 12 :257-261. DOI: 10.2174/138527208783497466 - 36.
Otto S, Bertoncin F, Engberts JBFN. Lewis acid catalysis of a Diels-Alder reaction in water. Journal of the American Chemical Society. 1996; 118 :7702-7707. DOI: 10.1021/ja960318k - 37.
Fringuelli F, Piermatti O, Pizzo F, Vaccaro L. Recent advances in Lewis acid catalyzed Diels-Alder reactions in aqueous media. European Journal of Organic Chemistry. 2001; 2001 :439-455. DOI: 10.1002/1099-0690(200102)2001:3%3C439::AID-EJOC439%3E3.0.CO;2-B - 38.
Kobayashi S, Manabe K. Development of novel Lewis acid catalysts for selective organic reactions in aqueous media. Accounts of Chemical Research. 2002; 35 :209-217. DOI: 10.1021/ar000145a - 39.
DeCastro C, Sauvage E, Valkenberg MH, Hölderich WF. Immobilised ionic liquids as Lewis acid catalysts for the alkylation of aromatic compounds with dodecane. Journal of Catalysis. 2000; 196 :86-94. DOI: 10.1006/jcat.2000.3004 - 40.
Moliner M. State of the art of Lewis acid-containing zeolites: Lessons from fine chemistry to new biomass transformation processes. Dalton Transactions. 2014; 43 :4197-4208. DOI: 10.1039/C3DT52293H