1. Introduction
The story of TLRs started with the discovery of Toll protein in the common fruit fly or
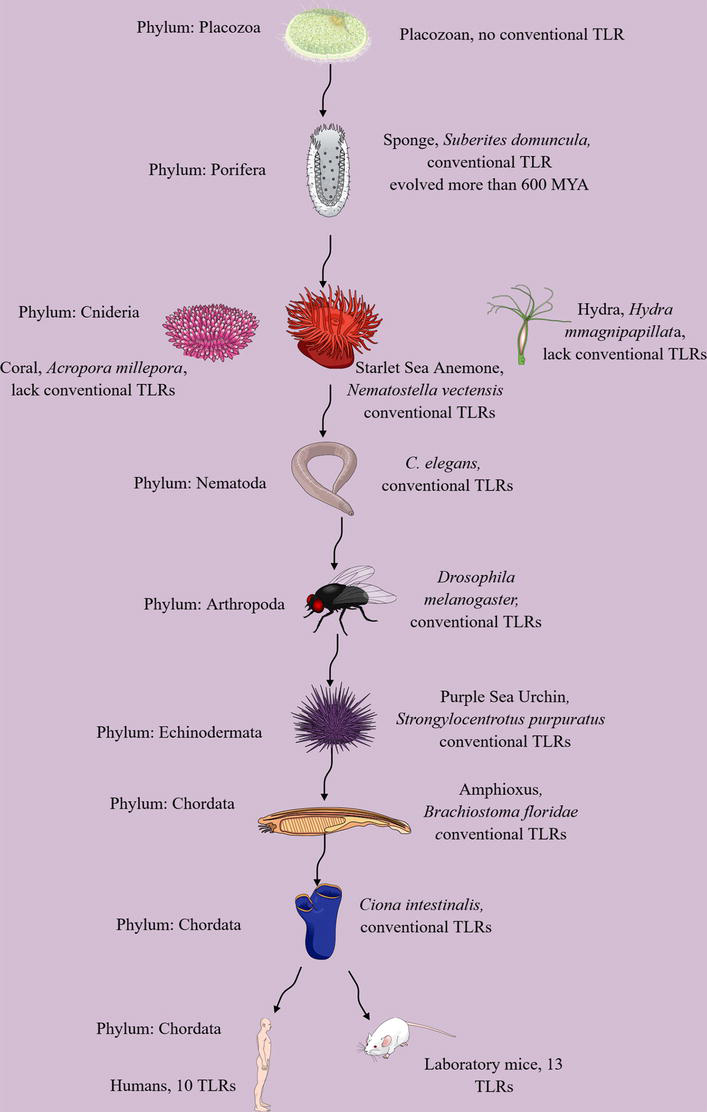
Figure 1.
Evolution of TLRs in the animal kingdom. Conventional TLRs are not present in placozoans, the smallest living animals. They first evolved in porifers or sponges more than 600 MYA. Thereafter, cnidarians, except Hydra and coral, express TLRs involved in host defense and development. Nematodes (
Evolution has played a critical role in the immune system’s development and function [22, 23]. TLRs are critical mediators of innate immune response against diverse pathogens and host-derived DAMPs. Their activation generates a pro-inflammatory immune response to central immune homeostasis and critically regulates adaptive immune response [18, 24, 25, 26, 27]. Hence, the current chapter introduces the evolution of TLRs, which is essential to understand as their dysregulation is associated with different inflammatory diseases and developmental defects.
2. Evolution of TLRs
Placozoans (
Starlet Sea anemones or
Notably, genomes of other cnidarians, such as Hydra (
The TOL-1 also regulates chemosensory Amphid Wing B (AWB) neurons sensing the cyclic lipodepsipentapeptide, Serrawettin W2, produced by
TLRs have not evolved due to coincidental evolution; instead, they originated due to multigene evolution, except for TLR5 and TLRS5, which may have evolved due to coincidental evolution [49]. Furthermore, they have evolved at a constant and conserved rate. Significant TLR families have diverged during or before the Cambrian period, which lasted for 53.4 million years from the end of the preceding Ediacaran Period 538.8 MYA to the beginning of the Ordovician Period 485.4 MYA and produced the most intense burst of evolution ever known [49]. Synonymous/non-synonymous substitution ratio evaluation has further not supported the positive selection pressure in the vertebrate phylogeny. The coding sequence, function, and signaling pathways initiated by vertebrate TLRs are highly conserved upon recognizing their corresponding ligands [49, 54, 55]. Hence, the TLRs are an example of the evolutionary conservation of a biological system at multiple levels, such as genes, proteins, and networks.
Further studies have indicated that the rapid speciation and adaptation to freezing water temperatures are not critical for the evolution of TLR numbers in Nototheniidae (Perciformes order, Notothenioidei sub-order). This stenothermal monophyletic teleost clade evolved relatively recently in the cold-stable waters of Antarctica). However, it induces a shift in the LRR pathogen recognition domain common to all the Nototheniidae analyzed, and of the six subfamilies of TLR in Nototheniidae fishes, TLR22 was most affected [56].
Furthermore, MyD88-dependent and MyD88-independent downstream TLR signaling pathways have evolved separately with common ancestors for vertebrate and invertebrate orthologs of the MyD88 adaptor molecule [57]. Thus, the MyD88 signaling pathway is very ancient as it originated in sponges), like TLRs, and early duplication events generated different adaptor molecules and their corresponding TLRs.
3. Conclusion
TLRs exist in sponges (Phylum Porifera), which have evolved at least 600 MYA. TLRs protected sponges from infections and critically regulated cellular (cnidocytes) development in cnidarians. They have carried over this ancestry to the hierarchy of the animal kingdom (humans). Hence, TLRs evolved in lower animals (Poriferans) to protect them from invading microbes.
References
- 1.
Anderson KV, Bokla L, Nüsslein-Volhard C. Establishment of dorsal-ventral polarity in the Drosophila embryo: The induction of polarity by the toll gene product. Cell. 1985; 42 :791-798 - 2.
Anderson KV, Jürgens G, Nüsslein-Volhard C. Establishment of dorsal-ventral polarity in the Drosophila embryo: Genetic studies on the role of the toll gene product. Cell. 1985; 42 :779-789 - 3.
Lemaitre B, Nicolas E, Michaut L, Reichhart JM, Hoffmann JA. The dorsoventral regulatory gene cassette spätzle/toll/cactus controls the potent antifungal response in Drosophila adults. Cell. 1996; 86 :973-983 - 4.
Shan T, Wang Y, Bhattarai K, Jiang H. An evolutionarily conserved serine protease network mediates melanization and toll activation in Drosophila. Science Advances. 2023; 9 :eadk2756 - 5.
Leulier F, Lemaitre B. Toll-like receptors–taking an evolutionary approach. Nature Reviews. Genetics. 2008; 9 :165-178 - 6.
Bell JK, Mullen GE, Leifer CA, Mazzoni A, Davies DR, Segal DM. Leucine-rich repeats and pathogen recognition in toll-like receptors. Trends in Immunology. 2003; 24 :528-533 - 7.
Bell JK, Botos I, Hall PR, Askins J, Shiloach J, Segal DM, et al. The molecular structure of the toll-like receptor 3 ligand-binding domain. Proceedings of the National Academy of Sciences of the United States of America. 2005; 102 :10976-10980 - 8.
Choe J, Kelker MS, Wilson IA. Crystal structure of human toll-like receptor 3 (TLR3) ectodomain. Science. 2005; 309 :581-585 - 9.
Watanabe T, Tokisue T, Tsujita T, Matsumoto M, Seya T, Nishikawa S, et al. N-terminal binding site in the human toll-like receptor 3 ectodomain. In: Nucleic Acids Symp Ser (Oxf). England: Oxford University Press; 2007. pp. 405-406 - 10.
Gay NJ, Keith FJ. Drosophila toll and IL-1 receptor. Nature. 1991; 351 :355-356 - 11.
Ghosh S, Gifford AM, Riviere LR, Tempst P, Nolan GP, Baltimore D. Cloning of the p50 DNA binding subunit of NF-kappa B: Homology to rel and dorsal. Cell. 1990; 62 :1019-1029 - 12.
O’Neill LA, Greene C. Signal transduction pathways activated by the IL-1 receptor family: Ancient signaling machinery in mammals, insects, and plants. Journal of Leukocyte Biology. 1998; 63 :650-657 - 13.
Medzhitov R, Preston-Hurlburt P, Janeway CA Jr. A human homologue of the Drosophila toll protein signals activation of adaptive immunity. Nature. 1997; 388 :394-397 - 14.
Kumar V. Toll-like receptors in immunity and inflammatory diseases: Past, present, and future. International Immunopharmacology. 2018; 59 :391-412 - 15.
Blasius AL, Beutler B. Intracellular toll-like receptors. Immunity. 2010; 32 :305-315 - 16.
Kumar V, Barrett JE. Toll-like receptors (TLRs) in health and disease: An overview. Handbook of Experimental Pharmacology. 2022; 276 :1-21 - 17.
Wu J, Chen ZJ. Innate immune sensing and Signaling of cytosolic nucleic acids. Annual Review of Immunology. 2014; 32 :461-488 - 18.
Kumar V. Toll-like receptors in the pathogenesis of neuroinflammation. Journal of Neuroimmunology. 2019; 332 :16-30 - 19.
Kawai T, Akira S. The role of pattern-recognition receptors in innate immunity: Update on toll-like receptors. Nature Immunology. 2010; 11 :373-384 - 20.
Lind NA, Rael VE, Pestal K, Liu B, Barton GM. Regulation of the nucleic acid-sensing toll-like receptors. Nature Reviews Immunology. 2022; 22 :224-235 - 21.
Janeway CA Jr. Approaching the asymptote? Evolution and revolution in immunology. Cold Spring Harbor Symposia on Quantitative Biology. 1989; 54 (Pt 1):1-13 - 22.
Flajnik MF, Kasahara M. Origin and evolution of the adaptive immune system: Genetic events and selective pressures. Nature Reviews Genetics. 2010; 11 :47-59 - 23.
Kaufman J. Evolution and immunity. Immunology. 2010; 130 :459-462 - 24.
Kumar V. Toll-like receptors in adaptive immunity. Handbook of Experimental Pharmacology. 2022; 276 :95-131 - 25.
Kumar V. Going, toll-like receptors in skin inflammation and inflammatory diseases. EXCLI Journal. 2021; 20 :52-79 - 26.
Kumar V. The trinity of cGAS, TLR9, and ALRs guardians of the cellular galaxy against host-derived self-DNA. Frontiers in Immunology. 2020; 11 :624597 - 27.
Kumar V. Toll-like receptors in sepsis-associated cytokine storm and their endogenous negative regulators as future immunomodulatory targets. International Immunopharmacology. 2020; 89 :107087 - 28.
Kamm K, Schierwater B, DeSalle R. Innate immunity in the simplest animals – Placozoans. BMC Genomics. 2019; 20 :5 - 29.
Orús-Alcalde A, Lu TM, Børve A, Hejnol A. The evolution of the metazoan toll receptor family and its expression during protostome development. BMC Ecology and Evolution. 2021; 21 :208 - 30.
Wiens M, Korzhev M, Perovic-Ottstadt S, Luthringer B, Brandt D, Klein S, et al. Toll-like receptors are part of the innate immune defense system of sponges (demospongiae: Porifera). Molecular Biology and Evolution. 2007; 24 :792-804 - 31.
Wiens M, Korzhev M, Krasko A, Thakur NL, Perović-Ottstadt S, Breter HJ, et al. Innate immune defense of the sponge Suberites domuncula against bacteria involves a MyD88-dependent signaling pathway. Induction of a perforin-like molecule. The Journal of Biological Chemistry. 2005; 280 :27949-27959 - 32.
Ohnishi H, Tochio H, Kato Z, Orii KE, Li A, Kimura T, et al. Structural basis for the multiple interactions of the MyD88 TIR domain in TLR4 signaling. Proceedings of the National Academy of Sciences. 2009; 106 :10260-10265 - 33.
Bonnert TP, Garka KE, Parnet P, Sonoda G, Testa JR, Sims JE. The cloning and characterization of human MyD88: A member of an IL-1 receptor related family. FEBS Letters. 1997; 402 :81-84 - 34.
Yin Z, Zhu M, Davidson EH, Bottjer DJ, Zhao F, Tafforeau P. Sponge grade body fossil with cellular resolution dating 60 Myr before the Cambrian. Proceedings of the National Academy of Sciences of the United States of America. 2015; 112 :E1453-E1460 - 35.
Sullivan JC, Kalaitzidis D, Gilmore TD, Finnerty JR. Rel homology domain-containing transcription factors in the cnidarian Nematostella vectensis. Development Genes and Evolution. 2007; 217 :63-72 - 36.
Brennan JJ, Messerschmidt JL, Williams LM, Matthews BJ, Reynoso M, Gilmore TD. Sea anemone model has a single toll-like receptor that can function in pathogen detection, NF-κB signal transduction, and development. Proceedings of the National Academy of Sciences of the United States of America. 2017; 114 :E10122-e10131 - 37.
Tucker RP, Adams JC. Chapter eight – Adhesion networks of cnidarians: A postgenomic view. In: Jeon KW, editor. International Review of Cell and Molecular Biology. USA: Academic Press; 2014. pp. 323-377 - 38.
Satake H, Sekiguchi T. Toll-like receptors of deuterostome invertebrates. Frontiers in Immunology. 2012; 3 :34 - 39.
Wolenski FS, Bradham CA, Finnerty JR, Gilmore TD. NF-κB is required for cnidocyte development in the sea anemone Nematostella vectensis. Developmental Biology. 2013; 373 :205-215 - 40.
Miller DJ, Hemmrich G, Ball EE, Hayward DC, Khalturin K, Funayama N, et al. The innate immune repertoire in cnidaria--ancestral complexity and stochastic gene loss. Genome Biology. 2007; 8 :R59 - 41.
Bosch TC, Augustin R, Anton-Erxleben F, Fraune S, Hemmrich G, Zill H, et al. Uncovering the evolutionary history of innate immunity: The simple metazoan Hydra uses epithelial cells for host defence. Developmental and Comparative Immunology. 2009; 33 :559-569 - 42.
Franzenburg S, Fraune S, Künzel S, Baines JF, Domazet-Lošo T, Bosch TCG. MyD88-deficient Hydra reveal an ancient function of TLR signaling in sensing bacterial colonizers. Proceedings of the National Academy of Sciences. 2012; 109 :19374-19379 - 43.
Tenor JL, Aballay A. A conserved toll-like receptor is required for Caenorhabditis elegans innate immunity. EMBO Reports. 2008; 9 :103-109 - 44.
Brandt JP, Ringstad N. Toll-like receptor Signaling promotes development and function of sensory neurons required for a C. Elegans pathogen-avoidance behavior. Current Biology. 2015; 25 :2228-2237 - 45.
Pradel E, Zhang Y, Pujol N, Matsuyama T, Bargmann CI, Ewbank JJ. Detection and avoidance of a natural product from the pathogenic bacterium Serratia marcescens by Caenorhabditis elegans. Proceedings of the National Academy of Sciences of the United States of America. 2007; 104 :2295-2300 - 46.
Kim DH. Signal transduction: A different kind of toll is in the BAG. Current Biology. 2015; 25 :R767-R769 - 47.
Pujol N, Link EM, Liu LX, Kurz CL, Alloing G, Tan MW, et al. A reverse genetic analysis of components of the toll signaling pathway in Caenorhabditis elegans. Current Biology. 2001; 11 :809-821 - 48.
Rast JP, Smith LC, Loza-Coll M, Hibino T, Litman GW. Genomic insights into the immune system of the sea urchin. Science. 2006; 314 :952-956 - 49.
Roach JC, Glusman G, Rowen L, Kaur A, Purcell MK, Smith KD, et al. The evolution of vertebrate toll-like receptors. Proceedings of the National Academy of Sciences. 2005; 102 :9577-9582 - 50.
Davidson CR, Best NM, Francis JW, Cooper EL, Wood TC. Toll-like receptor genes (TLRs) from Capitella capitata and Helobdella robusta (Annelida). Developmental and Comparative Immunology. 2008; 32 :608-612 - 51.
Rauta PR, Samanta M, Dash HR, Nayak B, Das S. Toll-like receptors (TLRs) in aquatic animals: Signaling pathways, expressions and immune responses. Immunology Letters. 2014; 158 :14-24 - 52.
Satake H, Sasaki N. Comparative overview of toll-like receptors in lower animals. Zoological Science. 2010; 27 :154-161 - 53.
Palti Y. Toll-like receptors in bony fish: From genomics to function. Developmental and Comparative Immunology. 2011; 35 :1263-1272 - 54.
Kim DH, Ausubel FM. Evolutionary perspectives on innate immunity from the study of Caenorhabditis elegans. Current Opinion in Immunology. 2005; 17 :4-10 - 55.
Phelan PE, Mellon MT, Kim CH. Functional characterization of full-length TLR3, IRAK-4, and TRAF6 in zebrafish (Danio rerio). Molecular Immunology. 2005; 42 :1057-1071 - 56.
Sousa C, Fernandes SA, Cardoso JCR, Wang Y, Zhai W, Guerreiro PM, et al. Toll-like receptor evolution: Does temperature matter? Frontiers in Immunology. 2022; 13 . DOI: 10.3389/fimmu.2022.812890 - 57.
Roach JM, Racioppi L, Jones CD, Masci AM. Phylogeny of toll-like receptor signaling: Adapting the innate response. PLoS One. 2013; 8 :e54156