The interaction distance, Eads and Fermi energy, Eg in gas phase of all TiO2 surface on organic additives.
Abstract
This chapter introduced a new series of organic compound additives like thiophene 2,5-dicarboxylic acid (TDA), sulfanilamide (SAA), 2,6-diamino pyridine (DAP), dibenzo-18-crown-6 (DBC) and 2,6-pyridine dicarboxylic acid (PDA) with gelatin/KI/I2 consist gel polymer electrolytes for dye-sensitized solar cells (DSSCs) application. Nowadays, it is focusing on biopolymers for preparing gel electrolytes for DSSCs application which is a conventional renewable energy source. Biopolymers are abundant in nature, and they are non-toxic, thermally stable, environmentally friendly, low-cost, and have good mechanical and physical properties. The introduced novel gelatin (GLN) biopolymer-based gel electrolytes play a role in improving ionic conductivity and stability, and it also play a better ability for ionic mobility. The low-cost and commercialized organic additive molecules with electron donors like S, O and N elements were strongly coordinated on the surface TiO2 and fermi level shift into negative potentials. The organic additive compound SAA achieved a very active additive and easily reduced the recombination reaction between the surface of TiO2 and I3− ions. This phenomenon readily improves the stability and overall η of the DSSC. During the DSSCs process, intrinsic charge carrier transfer between both electrodes as well as the continuous regeneration of the dye molecules. The surface study and conductivity of prepared gelatin-based gel electrolyte with N, S and O-based additives were characterized by fourier transform infrared spectroscopy (FTIR), UV-visible, X ray diifraction (XRD), Electrochemical Impedance Spectroscopy (EIS) and dye-sensitized solar cells (DSC), respectively. Furthermore, to examine the adsorption behaviour of organic additives on TiO2 (101) surface and negative fermi level shift on TiO2 surface were analysed by density functional theory (DFT) theoretical study.
Keywords
- DBA
- TDA
- DSSCs
- TiO2
- biopolymers
1. Introduction
DSSC has a conventional renewable energy source and ecologically interest owing to their simple design, easy fabrication, low production cost and high power conversion effiency (PCE) [1, 2]. O’Regan and Grätzel were the first to achieve PCE of 7.1% for DSSCs devices in 1991. Currently, DSSCs achieved a PCE 15.1% efficiency increase by using two metal-free organic dyes [3, 4]. In DSSCs device, there are three major constituents as listed below: (1) FTO plate coated using TiO2 nanocrystal, it will act as a photo anode as well as grip for dye molecules (sensitizers), (2) redox couple I−/I3− act as a charge transfer which contains electrolyte act as an oxidized dye molecule it regenerates continuously and (3) FTO plate act as a counter electrode which is regenerate the electrolyte using platinum coated. Photosensitizers yielding superior photocurrents are crucial for copper-electrolyte-based, highly efficient dye-sensitized solar cells (DSCs). One of the essential constituents in the DSSCs is the electrolyte, which has five main constitutes: (i) glass conductive substrate, (ii) sensitizing dye, (iii) an electrolyte containing a redox couple, (iv) a semiconductor, and (v) a platinized counter electrode. DSSCs have a combination of the combination which affects the performance dramatically [5, 6]. DSSCs failed to propose the liquid electrolytes there are numerous reports based on polymer electrolytes. During the DSSCs process, intrinsic charge carrier transfer between both electrodes as well as the continuous regeneration of the dye molecules. One of the important factors that affect the photovoltaic performance of DSSCs is a solvent type and immersion time for the photoanode. To prepare the solvent chosen for the photo anode affects the adsorption of the dye on the surface of a semiconductor. This liquid electrolyte has some disadvantages, like liquid volatilization, leakage and sealing complications, and voltage fluctuation which have a significant impact on the long-term stability, maintenance and performance of the DSSCs [7, 8, 9, 10]. The polymeric chain diffusion of ions by intermolecular interaction would alter the ionic conductivity of the polymer. The blending technique helps to reduce the enhancement of the dielectric constant, the energy barrier of charge carrier ions, subdues melting point, boiling point and viscosity. The advantages of blended gel polymer electrolytes such as better charge carrier mobility, tensile strength, high thermal and chemical stability, flexibility, good fluidity, less spillage, excellent dielectric constant, increasing ionic conductivity and ion transfer rate. The gel-based polymer electrolytes enhanced the long-term stability in DSSCs [11, 12]. Several researchers have prepared gel electrolytes have been used. Polymers such as poly (acrylonitrile), poly (vinyl chloride), poly (acrylonitrile), poly (propylene oxide), poly (ethylene oxide), poly (vinylidene fluoride hexafluoropropylene), poly (vinylidene fluoride), poly (methyl methacrylate), etc., used in DSSCs applications [13, 14, 15, 16, 17, 18]. Biopolymers are abundant in nature, and they are non-toxic, thermally stable, environmentally friendly, low-cost and include good mechanical and physical properties [19].
Researchers are currently focusing on biopolymers for prepared gel electrolytes to DSSCs application, such as
2. Experimental section
2.1 Preparation of GLN gel electrolytes
Firstly, gelatin 0.30 g was dissolved in glycerol solvent. Then iodine 0.010 g and potassium iodide 0.030 g were mixed in DMF solvent and the mixture was kept in magnetic stirring. The whole mixture electrolyte was stirred continuously at 3 hours for 70°C. After 3 hours stirred, we get gel mixture (dark brown colour).
The glycerol solvent was extra added until to the gelatin polymer 0.30 g dissolved and then additionally added iodine 0.010 g, potassium iodide 0.030 g, SAA 0.010 g in DMF solvent. Afterwards, the whole combination was magnetic stirred well at 3 hours for 70°C to get gel formation. The same procedure was followed for all another organic additive like PDA, TDA, DBC and DAP other gel electrolytes. The selected commercial organic additives structure and photographs of the six gel electrolytes given in Figures 1 and 2.

Figure 1.
Chemical name and structure of selected organic additives.

Figure 2.
Images of gel electrolytes without and with additives.
3. Results and discussion
The XRD result of the gel electrolytes was shown in Figure 3. Previous literature, the gelatin polymer exhibited broadening peak at 2θ range, 22.35° [41]. From the XRD pattern showed broad peaks around 22° was observed for the entire gel polymer by the amorphous nature of the gel electrolytes which further proved the high conducting electrolyte behaviour. Further it confirms that the organic additives, co-ordinates well with the gelatin polymers matrix and redox couple of gel polymer sample resourcefully. The amorphous phase of such gelatin gel polymer samples is notable parameter in expressing the conductivity nature. Hence, it shows that the conductivity increased with the amorphous nature.

Figure 3.
XRD pattern of gel electrolytes without and with additives.
The gelatin gel electrolytes with/without organic additives added upon were measured by FTIR with 400 to 4000 cm−1 range. The corresponding FTIR spectroscopy specified in Figure 4. From the FTIR, clearly exposes the vibration and stretching frequency of all the gelatin gel electrolyte with/without additives. The broad peak was observed at 3500–3200 cm−1 assigned for biopolymer gelatin hydroxyl group. Due to C = O vibrational, stretching frequency intensity peak was attained highly at 1640 cm−1. N-H bending allocated at 1530 cm−1 is sharp peak. Small intensity peak was attributed at 1445–1340 cm−1 owing to the bending vibration of aliphatic C-H group and noted C-N stretching frequency showed at 1235 cm−1 [42]. FTIR clearly shows that the inclusion of organic additives in gelatin gel electrolyte with composition of KI/I2 changes a small intensity shift, which shows organic additives and redox pair well connection with gelatin polymer.

Figure 4.
FTIR spectroscopy of gel electrolytes without and with additives.
The gelatin gel electrolyte absorbance range was investigated by UV-visible spectroscopy. Figure 5 describes the GLN gel electrolyte without additive absorbance range was shown at 313 nm. The gelatin gel electrolytes with organic additives SAA, TDA, PDA, DAP and DBC correspond to 293, 290, 296, 292 and 295 nm, respectively. This small changes in UV-visible absorption may be due to the dissimilar functional groups present in the organic additives interacting with gelatin gel electrolytes. Also, the UV-visible study confirmed the addition of organic additives well incorporated with the I3− ion in the gelatin polymer electrolyte. It exhibited a peak at 320-370 nm for all the organic additive-based gel electrolytes. This phenomenon increases the I3− ion mobility in the gelatin polymer electrolyte and enhanced well the DSSCs performances.

Figure 5.
UV-visible spectroscopy of gelatin gel electrolytes without and with additives.
The morphology structure studied about with and without organic additive in the gelatin gel electrolytes. Without organic additive GLN gel electrolyte given in Figure 6a, which showed the surface morphology like fibre nature and without space formation. Gelatin gel electrolyte with organic additives SAA (Figure 6b), TDA (Figure 6c), PDA (Figure 6d), DAP (Figure 6e) and DBC (Figure 6f) displayed surface morphology like fibre and with space formation which confirms the addition of additives changed the surface of the gelatin gel electrolyte. From the scanning electron microscopy (SEM), it clearly observed that gelatin and additive gel electrolytes increase the amorphous nature and improve the ionic conductivity in DSSCs.

Figure 6.
SEM images of gelatin gel electrolytes (a) GLN, (b) SAA, (c) TDA, (d) PDA, (e) DAP and (f) DBC.
DSC study for gelatin gel electrolytes with and without additives was caried under an annealing temperature of 50 to 300°C with nitrogen atmosphere at 10 k/min. The Figure 7 displayed decomposition peak for without and with organic additive gel electrolyte. GLN peak revealed at 230°C, which is without additive gel electrolyte. The organic additives-based gel electrolyte exhibited decomposition peak at 231°C (SAA), 233°C (TDA), 208°C (PDA), 234°C (DAP) and 241°C (DBC), respectively. The variation of the decomposition may be due to the different organic additives and KI/I2 incorporated into the gelatin gel electrolytes. The broadening decomposition peak confirms the nature of the amorphous gel electrolytes owing to interfacial interaction between the organic additives and polymer. These thermal results prove the gelatin gel electrolytes have a good stability in DSSC performances.

Figure 7.
DSC curves of gelatin gel electrolytes without and with additives.
All the organic additives were optimized at a gas phase by Gussian16, and optimized geometries of organic additives compound are shown in Figure 8. Highest occupied molecular orbital (HOMO)-lowest unoccupied molecular orbital (LUMO) orbital calculated for the organic additives done by frontier molecular orbital (FMO) analysis optimization at gas phase. Figure 9 shows the HOMO-LUMO for organic additive compounds. Figure 9 observes the electronic shift and charge transfer in different orbitals. The transition occurs primarily from the aromatic cloud towards the functional group present in it, which has been noted. The additives containing carboxylic acid and amino functional groups have been mostly noticed. In DBC additive, it has the least efficiency due to the absence of these functional groups, and the orbitals are only localized at the π ring due to no transition between HOMO and LUMO. Table 1 shows the Energy Gap (Eg) for all the organic additives between HOMO and LUMO.

Figure 8.
Optimized geometries of additive compounds at PBE/6–311++G**.

Figure 9.
HOMO and LUMO orbitals calculated for different organic additives in gas phase.
Sample code | Eg in gas phase (eV) | Shortest interaction distances (Additive·TiO2 Surface) | Eads (kcal/mol) | Fermi energy (eV) | |
---|---|---|---|---|---|
Covalent bond (Å) | Non-covalent (Å) | ||||
SAA | 3.82 | 2.08 (Ti-O) 2.27 (Ti-N) | 1.67 (N-H···O) | −105.88 | −3.82 |
TDA | 3.31 | 1.85 – 1.93 (Ti-O) | 1.65 –1.95(O-H+···O) | −368.53 | −3.88 |
PDA | 3.32 | 1.89 – 2.24 (Ti-O) | 1.70 (O-H+···O) | −202.35 | −3.89 |
DAP | 3.63 | 2.05 – 2.26 (Ti-N) | 1.55 – 1.76 (N-H···O) | −225.62 | −3.60 |
DBC | 4.07 | — | 2.15 – 2.98 (vdWs) | −69.74 | −3.16 |
Table 1.
(a), (b) represents the single and double proton transfer from carboxylate group of additives into TiO2 surface, respectively. (c), (d) represents the van der Waals interaction distances and hydrogen bonding, respectively.
Eg from the table shown that HOMO-LUMO gap is a high for DBC additive. The FMOs are mainly referred to as the lowest unoccupied molecular orbital (LUMO) highest occupied molecular orbital (HOMO). The photosensitizer for FMOs should be aligned appropriately with the other main two components in the DSSC, i.e., the electrolyte shuttle and semiconductor. The conduction band (CB) of the semiconductors is less stable (destabilized) with respect to LUMO. The order of Eg for all the organic additives as follows DBC > SAA > DAP > PDA > TDA. In the DSSCs process, the entire organic additive HOMO-LUMO gap has a great impact on the transferred electron [28]. The charge transfer process will be more difficult, if the molecule has a higher Eg. In this case, the molecule with the greatest Eg (i.e., DBC), when compared to others has the least efficiency. As a result, it is evident that the Eg plays a very important role in the PCE of DSSCs in the additive molecule.
The gas phase optimization for additives optimized on the TiO2 (101) surface. All of these total phase DFT calculations was performed using the CP2K code. During the optimization, the upper two layers were free and lowest two layers was kept fixed surface. After the optimization, the interaction distances, fermi energies, Eads of the additives were calculated and the values given in Table 1. The interaction distance between the organic additives and anatase TiO2 surface have strong non-covalent bonds and covalent bond (i.e., H-bond and vdWs) interactions. The calculated fermi energy and interaction distance are displayed in Table 1. Closer analysis of the distance reveals more efficient combinations of strong Ti-O and Ti-N bonds with H-bonded (~ 1.8 to 2.3 Å) interaction on the surface. The strong interaction between the additives and TiO2 surface is important and significant in the efficiency of the complexes. The shortest H-bond distance and strong covalent bond (1.65 and 1.67 Å) of additive SAA and TDA compounds showed the highest PCE of 5.8 and 5.2%. Due to the formation of covalent bond interaction in PDA and TDA carboxylates encourage the proton transferred into the TiO2 surface. This DAP additive may be stabilized by two H-bonds (N-H·····O) and Ti-N bonds between the DAP and TiO2. It is also exhibited an efficiency of 4.3%, which is lower efficiency than the other (SAA, PDA and TDA) additives remarkable in this study.
Previous literature exposes that nature of the geometries and interaction act as vital role in PCE of the DSSCs device [43, 44]. Different functional groups present in all five organic additives strongly interact with covalent and non-covalent (H-bond and vdWs) on TiO2 surface and it was clearly revealed the Figure 9. The carboxylic acid and amino functional group performance has a major significance in stimulating the electrostatic interaction between the organic additives and surface TiO2. These dealings have a gigantic effect on the charge transfer and PCE of DSSCs. The strong interaction can be computed from Eads values. The organic additives Eads values −105.9 (SAA), −368.5 (TDA), −225.6 kcal/mol (DAP) and − 202.4 (PDA), respectively. Due to the anchoring bond strongly present in between the functional groups in additive and TiO2 surface, it is exciting to note that the carboxylic functional group present in the TDA and PDA denote the proton from organic additive to TiO2 surface. There is a strong H-bond formation between the carboxylate anion and TiO2 (OH) which indicates the ionic state. Thus, these organic additives are powerfully bound to boost the charge transfer and TiO2 surface, which directly impacts the PCE of DSSCs. The less DBC Eads (−69.7 kcal/mol) which attributed to weak vdWs interaction in the absence of anchoring groups with TiO2 surface. DBC additive has a no robust electrostatic interaction like other organic additives, which is obviously from distances and interaction sites (Figure 9) when compared to other organic additives this is due to the least efficiency of DBC additive. Furthermore, the energy of fermi is not that much affected by different additive molecules. The low fermi energy (≈ 3.5 eV) of the complexes helps in the easy transfer of the charge in DSSCs. After the DFT studies, it was clearly understood that the strong interaction between the organic additive functional groups and surface TiO2 has important role to act in charge transfer process and hence in improved PCE of DSSCs.
The entire gel electrolyte without and with additives was studied via EIS (Electrochemical Impedance Spectroscopy) under room temperature by the ionic conductivity of. All the measured gel electrolytes scan range from 300 KHz to 400 MHz (0.800 V) as single sign mode and to set Ewe to E. The ionic conductivity of gel electrolytes were calculated from Nyquist plot. The conductivity of gel electrolytes in the order as follows SAA (2.93

Figure 10.
DFT optimized geometries of organic additives on TiO2 (101) surface with shortest interacting distances (Å).

Figure 11.
Nyquist plot of gel electrolytes without and with additives.
EIS study was examined for photo electrochemical process such as resistance and conductance during DSSCs process. This study mainly discussed about the interfacial charge transfer in DSSCs like charge transfer between the photo anode/electrolyte and platinum/electrolyte reactivity termed as Cμ, Rct and Rpt [45]. The EIS data received for devices under darkness was fitted by Zfit software. Rs (R1), Rpt (R2), Rct (R3) and Cμ (C3) values collected from fitting equivalent circuit and the values tabulated in Table 2. The Rs, Rpt, Rct and Cμ corresponds to series resistance, charge transfer between electrolytes and photoelectrodes interface and chemical capacitance of the DSSCs device. Figures 12 and 13 display chemical capacitance and charge transfer for gel electrolytes. The SAA additive gel electrolyte shows low Rpt value of 685 Ω, high Rct of 1674 Ω and Cμ of 5.702
Electrolyte system | R1 Rs (Ω) | R2 Rpt (Ω) | R3 Rct (Ω) | C3 Cμ (F) | σ (S/cm−1) | τn (ms) |
---|---|---|---|---|---|---|
SAA/I−/I3−/gelatin | 685 | 685 | 1674 | 5.702×10−6 | 2.93×10−5 | 6.6 |
TDA/I−/I3−/gelatin | 705 | 684 | 1024 | 4.926×10−6 | 2.85×10−5 | 4.5 |
PDA/I−/I3−/gelatin | 727 | 727 | 689 | 4.861×10−6 | 2.77×10−5 | 4.0 |
DAP/I−/I3−/gelatin | 749 | 762 | 462 | 4.842×10−6 | 2.67×10−5 | 3.1 |
DBC/I−/I3−/gelatin | 840 | 1478 | 446 | 4.780×10−6 | 2.38×10−5 | 3.0 |
I−/I3−/gelatin(GLN) | 1172 | 3623 | 411 | 4.294×10−6 | 1.72×10−5 | 2.6 |
Table 2.
Electron life time and ionic conductivity values of gel electrolytes without and with additives.

Figure 12.
Conductivity variation of gel electrolytes with and without additives.

Figure 13.
Chemical capacitance of gel electrolytes without and with additives.
The without additive GLN gel electrolyte displayed high Rpt value of 3623 Ω, low Rct of 411 Ω and Cμ of 4.294
The chemical capacitance and charge transfer resistance results revealed that organic additives are an important key function of complex formation with I3− ions. Furthermore, in the fermi level of TiO2, it act on the TiO2 surface caused an immediate change, thereby the charge for the recombination processes get reduced. The augmented chemical capacitance after the addition of additive SAA combined with gel gelatin electrolyte, when the recombination process get decreased for production of good PCE.
Electron life time derived from bode phase plot which was experimental result of EIS measurement and bode phase graph given in Figure 14. The recombination kinetics between the photoanode and electrolytes interfaces are relevant to the maximum peak frequency (fmax) value from the bode phase plot of gel electrolytes. Electrolytes and electrodes interfaces electron lifetime was calculated by following derivation [46].
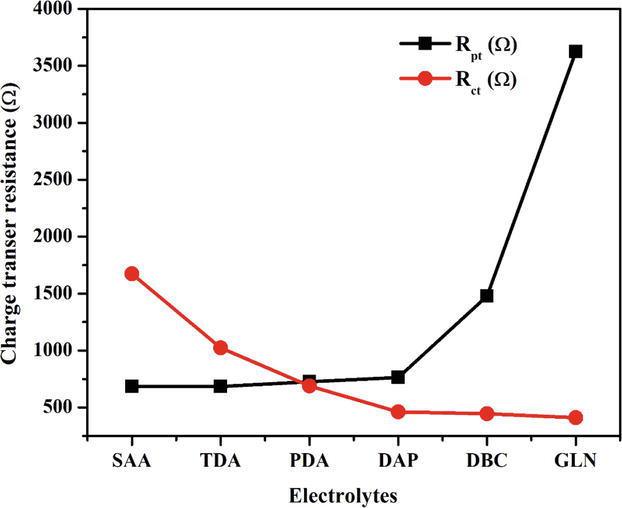
Figure 14.
Charge transfer resistance of gel electrolytes without and with additives.
The τn values of gel electrolytes given in Table 2. The τn of gel electrolytes variation of each other owing to presence of various functional groups present in organic additives. The SAA additive incorporated with gel electrolyte showed a high τn (6.6 ms) which compared with other organic additives-based gel electrolyte. The without additive GLN gel electrolyte exhibited low τn of 2.6 ms. From the τn results, increment of τn of SAA gel electrolyte showed reduced recombination process among TiO2 surface and I3− ions. By modifying the components, we were able to find out with and without the addition of gel electrolyte using DSSCs device, and used for their optical, electrochemical and photovoltaic characteristics [47, 48, 49].
I-V measured for gel electrolytes without and with additives was carried by 1.5 Am with sun illumination of 100 mWcm−2 and I-V curves are given in Figure 14. The photovoltaic results of Voc, FF, Jsc and η presented in Table 3. The SAA additive gel electrolyte act as a greater electrolyte in DSSCs, which showed the higher PCE of 5.8%. This SAA organic additive has electron-rich donor atoms combined in a structure such as N, O and S, and it stimulates the redox transfer of ions in gel electrolyte via complex formation. Therefore, TiO2 fermi level has less negative shift evidently proved to be improve the PCE of DSSCs device. Without additive GLN gel electrolyte attained PCE of 3.9% because of GLN gel electrolytes have a less conductivity and charge transfer process. After that, the addition of organic additives into the gel electrolytes improves the PCE DSSCs device. The variation of structure and functional groups in organic additives improves the amorphous nature of gelatin polymer and the good diffusion pathway of I3− ions in the gel nature.
Electrolyte system | Jsc (mA cm−2) | Voc (V) | Fill factor (FF) | Efficiency (η) |
---|---|---|---|---|
SAA/ I−/ I3−/gelatin | 14.1 | 790 | 0.52 | 5.8 |
TDA/ I−/ I3−/gelatin | 13.0 | 780 | 0.51 | 5.2 |
PDA/ I−/ I3−/gelatin | 12.4 | 770 | 0.51 | 4.9 |
DAP/ I−/I3−/gelatin | 11.3 | 765 | 0.51 | 4.4 |
DBC/I−/ I3−/gelatin | 11.0 | 760 | 0.51 | 4.3 |
I−/ I3−/gelatin | 10.1 | 752 | 0.51 | 3.9 |
Table 3.
I-V results of gel electrolytes without and with additives for DSSCs device under 1 sun illumination (100 mWcm2).
The device stability of the gel electrolyte without and with additives was assigned for the stability test at room temperature and its stands for 6 days (Figure 15). The calculated efficiency by Voc, FF, Jsc and η (Figure 16).

Figure 15.
I-V curve of gel electrolytes without and with additives.

Figure 16.
Stability graph of gel electrolytes without and with additives.
4. Conclusion
In this study, good stability and high performance of DSSCs device due to inexpensive incorporation of organic additives with rich lone pair N, O and S groups into gel electrolytes biopolymer gel which compared to without organic additive gel electrolyte. The organic additives (i.e., SAA, PDA, TDA, DBC and DAP) were linked in the surface of TiO2 that readily fermi level shifts in CB of TiO2 to a negative potential. The integration of S, O and N-containing organic additives into the gel electrolytes improves the ionic conductivity from 1.72
References
- 1.
Chowdhury FI, Buraidah MH, Arof AK, Mellander BE, Noor IM. Impact of tetrabutylammonium, iodide and triiodide ions conductivity in polyacrylonitrile based electrolyte on DSSC performance. Solar Energy. 2020; 196 :379-388 - 2.
Prajapat K, Dhonde M, Sahu K, Prateek B, Murty VVS, Shirage PM. The evolution of organic materials for efficient dye-sensitized solar cells. 2023; 55 :100586 - 3.
Peng JD, Wu YT, Yeh MH, Kuo FY, Vittal R, Ho KC. Transparent cobalt selenide/graphene counter electrode for efficient dye-sensitized solar cells with Co2+/3+-based redox couple. ACS Applied Materials & Interfaces. 2020; 12 (40):44597-44607 - 4.
Sen A, Putra MH, Biswas AK, Behera AK, Gro A. Insight on the choice of sensitizers/dyes for dye sensitized solar cells. 2023; 213 :111087 - 5.
Masud, Kim HK. Redox shuttle-based electrolytes for dye-sensitized solar cells: Comprehensive guidance, recent progress, and future perspective. ACS Omega. 2023; 8 (7):6139-6163 - 6.
Grobelny A, Shen Z, Eickemeyer FT, Antariksa NF, Zapotoczny S, Zakeeruddin SM, et al. A molecularly tailored photosensitizer with an efficiency of 13.2% for dye-sensitized solar cells. Advanced Materials. 2023; 35 (5):2207785 - 7.
Arslan BS, Öztürk N, Gezgin M, Sevindik S, Yılan D, Kumbasar RA, et al. Optimization of fabrication parameters for efficient dye-sensitized solar cells based on dyes containing benzothiadiazole, indoline, and their co-sensitization. Electrochimica Acta. 2023; 184 :142523 - 8.
Santhosh SG, Balamurugan S. Novel indole-based photosensitizers coupled with PEG-HEC quasi-solid-state electrolyte to improve energy conversion and stability of organic dyes based-dye sensitized solar cells. 2021; 389 :138771 - 9.
Krishna J, G, Ojha PK, Kar S, Roy K, Leszczynski J. Chemometric modeling of power conversion efficiency of organic dyes in dye sensitized solar cells for the future renewable energy. 2020; 69-70 :10453 - 10.
Bashir MBA, Rajpar AH, Salih EY, Ahmed EM. Preparation and photovoltaic evaluation of CuO@Zn(Al)O-mixed metal oxides for dye sensitized solar. Cell Nanomaterials. 2023; 13 (5):802 - 11.
Alizadeh A, Roudgar-Amoli M, Bonyad-Shekalgourabi S-M, Shariatinia Z, Mahmoudi M, Saadat F. Dye sensitized solar cells go beyond using perovskite and spinel inorganic materials: A review. Renewable and Sustainable Energy Reviews. 2022; 157 :112047 - 12.
Wategaonkar SB, Parale VG, Pawar RP, Mali SS, Hong CK, Powar RR, et al. Structural, morphological, and optical studies of hydrothermally synthesized Nb-added TiO2 for DSSC application. Ceramics International. 2021; 47 :25580-25592 - 13.
Geleta TA, Imae T. Nanocomposite photoanodes consisting of p-NiO/n-ZnO heterojunction and carbon quantum dot additive for dye-sensitized solar cells. ACS Applied Nano Materials. 2022; 4 :236-249 - 14.
Alabdan HI, Alwabsi A, Alsagrey AM, AlKhulaif FH, Abdelgawad ME, AlQahtani NJ, et al. Usage of CuO in dye-sensitized solar cells and CuO-based dyesensitized solar cells: A review. IEEE Journal of Photovoltaics. 2022; 12 :1445-1452 - 15.
Chalkias DA, Giannopoulos DI, Kollia E, Petala A, Kostopoulos V, Papanicolaou GC. Preparation of polyvinylpyrrolidone-based polymer electrolytes and their application by in-situ gelation in dye-sensitized solar cells. Electrochimica Acta. 2018; 271 :632-640 - 16.
Salih EY, Bashir MBA, Rajpar AH, Badruddin IA, Bahmanrokh G. Rapid fabrication of NiO/porous Si film for ultra-violate photodetector: The effect of laser energy. Microelectronic Engineering. 2022; 258 :111758 - 17.
Li C, Xin C, Xu L, Zhong Y, Wu W. Components control for high-voltage quasi-solid state dye-sensitized solar cells based on two-phase polymer gel electrolyte. Solar Energy. 2019; 181 :130-136 - 18.
Abisharani JM, Dineshkumar R, Devikala S, Arthanareeswari M, Ganesan S. Influence of 2, 4-diamino-6-phenyl-1-3-5-triazine on bio synthesized TiO2 dye-sensitized solar cell fabricated using poly (ethylene glycol) polymer electrolyte. Materials Research Express. 2020; 7 (2):025507 - 19.
Kotatha D, Hirata M, Ogino M, Uchida S, Ishikawa M, Furuike T, et al. Preparation and characterization of electrospun gelatin nanofibers for use as nonaqueous electrolyte in electric double-layer capacitor. Journal of Nanotechnology. 2019 - 20.
Salih EY, Ramizy A, Aldaghri O, Mohd Sabri MF, Madkhali N, Alinad T, et al. In-depth optical analysis of Zn (Al) O mixed metal oxide film-based Zn/Al-layered double hydroxide for TCO application. Crystals. 2022; 12 :79 - 21.
Bashir MBA, Salih EY, Sabri MFM, Rajpar AH, Badruddin IA, Hussein MZ, et al. In-depth thermal, microstructural and photoluminescence analysis of mesoporous ZnO/ZnAl2O4-MMO: The effect of molar ratio. ECS Journal of Solid State Science and Technology. 2021; 10 :106006 - 22.
Salih EY, Sabri MFM, Tan ST, Sulaiman K, Hussein MZ, Said SM, et al. Preparation and characterization of ZnO/ZnAl2O4-mixed metal oxides for dye-sensitized photodetector using Zn/Al-layered double hydroxide as precursor. Journal of Nanoparticle Research. 2019; 21 :1-12 - 23.
Balamurugan S, Ganesan S. Novel cobalt redox materials admitted in natrosol polymer with a thiophene based additive as a gel polymer electrolyte to tune up the efficiency of dye sensitized solar cells. Electrochimica Acta. 2020; 329 :135169 - 24.
Qin H, Owyeung RE, Sonkusale SR, Panzer MJ. Highly stretchable and nonvolatile gelatin-supported deep eutectic solvent gel electrolyte-based ionic skins for strain and pressure sensing. Journal of Materials Chemistry C. 2019; 7 (3):601-608 - 25.
Liu S, Liu J, Wang T, Wang C, Ge Z, Liu J, et al. Preparation and photovoltaic properties of dye-sensitized solar cells based on zinc titanium mixed metal oxides. Colloids and Surfaces A: Physicochemical and Engineering Aspects. 2019; 568 :59-65 - 26.
Foruzin LJ, Rezvani Z, Nejati K. TiO2@NiAl-Layered double oxide nanocomposite: An excellent photoanode for a dye sensitized solar cell. Solar Energy. 2019; 186 :106-112 - 27.
Ge Z, Zhu Y, Wang C, Xia L, Guo L, Wu Y, et al. Investigation of the photoanode based on graphene/zinc aluminum mixed metal oxide for dye-sensitized solar cell. Journal of Sol-Gel Science and Technology. 2020; 95 :432-438 - 28.
Fasolini A, Sangiorgi N, Brandi ET, Sangiorgi A, Mariani F, Scavetta E, et al. Increased efficiency and stability of dye-sensitized solar cells (DSSC) photoanode by intercalation of Eosin Y into Zn/Al layered double hydroxide. Applied Clay Science. 2021; 212 :106219 - 29.
Tan CY, Omar FS, Saidi NM, Farhana NK, Ramesh S, Ramesh K. Optimization of poly (vinyl alcohol-co-ethylene)-based gel polymer electrolyte containing nickel phosphate nanoparticles for dye-sensitized solar cell application. Solar Energy. 2019; 178 :231-240 - 30.
Pullanjiot N, Swaminathan S. Enhanced electrochemical properties of metal oxide interspersed polymer gel electrolyte for QSDSSC application. Solar Energy. 2019; 186 :37-45 - 31.
Yang Y, Zhang Z, Gao J, Pan D, Yuan B, Guo X, et al. Metal-organic materials as efficient additives in polymer electrolytes for quasi-solid-state dye-sensitized solar cells. Journal of Alloys and Compounds. 2017; 726 :1286-1294 - 32.
Chang WC, Sie SY, Yu WC, Lin LY, Yu YJ. Preparation of nano-composite gel electrolytes with metal oxide additives for dye-sensitized solar cells. Electrochimica Acta. 2016; 212 :333-342 - 33.
Mazloum-Ardakani M, Arazi R, Haghshenas M, Tamaddon F, Alizadeh M. Synthesis of 2-amino-4-(4-(methylamino) phenyl)-6-phenylnicotinonitrile as a new additive for the passivation of the TiO2 surface and retarding recombination in dye-sensitized solar cells. Electrochimica Acta. 2018; 266 :452-459 - 34.
Nath NCD, Jun Y, Lee JJ. Guanidine nitrate (GuNO3) as an efficient additive in the electrolyte of dye-sensitized solar cells. Electrochimica Acta. 2016; 201 :151-157 - 35.
Riaz R, Ali M, Maiyalagan T, Arbab AA, Anjum AS, Lee S, et al. Activated charcoal and reduced graphene sheets composite structure for highly electro-catalytically active counter electrode material and water treatment. International Journal of Hydrogen Energy. 2020; 45 :7751-7763 - 36.
Bagheri O, Dehghani H, Afrooz M. Pyridine derivatives; new efficient additives in bromide/tribromide electrolyte for dye sensitized solar cells. RSC Advances. 2015; 5 (105):86191-86198 - 37.
Abraham N, Unni C, Philip D. Studies on bandgap tuning of visible light active heterojunction CuO/ZnO nanocomposites for DSSC application. Journal of Materials Science: Materials in Electronics. 2019; 29 :21002-21013 - 38.
Rajakumar P, Satheeshkumar C, Ravivarma M, Ganesan S, Maruthamuthu P. Enhanced performance of dye-sensitized solar cell using triazole based phenothiazine dendrimers as additives. Journal of Materials Chemistry A. 2013; 1 (44):13941-13948 - 39.
Godlewski MM, Kaszewski J, Kielbik P, Olszewski J, Lipinski W, Slonska-Zielonka A, et al. New generation of oxide-based nanoparticles for the applications in early cancer detection and diagnostics. Nanotechnology Reviews. 2020; 9 :274-302 - 40.
Salih EY, Ramizy A, Aldaghri O, Sabri MFM, Madkhali N, Alinad T, et al. Rapid synthesis of hexagonal-shaped Zn (Al) O-MMO nanorods for dye-sensitized solar cell using Zn/Al-LDH as precursor. Nanomaterials. 2022; 12 :1477 - 41.
Musa A, Ahmad MB, Hussein MZ, Saiman MI, Sani HA. Effect of gelatin-stabilized copper nanoparticles on catalytic reduction of methylene blue. Nanoscale Research Letters. 2016; 11 (1):1-13 - 42.
Zhuang C, Tao F, Cui Y. Anti-degradation gelatin films crosslinked by active ester based on cellulose. RSC Advances. 2015; 5 (64):52183-52193 - 43.
Karthika P, Ganesan S, Arthanareeswari M. Low-cost synthesized organic compounds in solvent free quasi-solid state polyethyleneimine, polyethylene glycol based polymer electrolyte for dye-sensitized solar cells with high photovoltaic conversion efficiencies. Solar Energy. 2018; 160 :225-250 - 44.
Karthika P, Ganesan S, Kamalakannan S, Prakash M. Design and synthesis of the D−π–A-structured coadsorbents with the phenanthraquinone core and its application in dye-sensitized solar cells. The Journal of Physical Chemistry C. 2020; 124 (18):9886-9899 - 45.
Pavithra N, Velayutham D, Sorrentino A, Anandan S. Poly (ethylene oxide) polymer matrix coupled with urea as gel electrolyte for dye sensitized solar cell applications. Synthetic Metals. 2017; 226 :62-70 - 46.
Selvaraj B, Shanmugam G, Kamaraj S, Gunasekeran A, Sambandam A. Effect of 1-substituted 2-(pyridin-2-yl)-1 H-Benzo [d] imidazole ligand-coordinated copper and cobalt complex redox electrolytes on performance of Ru (II) dye-based dye-sensitized solar cells. Inorganic Chemistry. 2021; 60 (3):1937-1947 - 47.
Nagaraj P, Sasidharan A, David V, Sambandam A. Effect of cross-linking on the performances of starch-based biopolymer as gel electrolyte for dye-sensitized solar cell applications. Polymers. 2017; 9 (12):667 - 48.
Khan A, Alamry KA. Recent advances of emerging green chitosan- based biomaterials with potential biomedical applications: A review. Carbohydrate Research. 2021; 506 (5):108-368 - 49.
Marques ADS, Da Silva VAS, Ribeiro ES. Dye-sensitized solar cells: Components screening for glass substrate, counter-electrode, photoanode and electrolyte. Materials Research. 2020; 23 (5):e20200168