Abstract
Neurofeedback (NFB) is a closed-loop technique in which the patient receives feedback on brain activity to encourage voluntary control of brain activity. NFB promotes neuroplasticity and changes the brain functionally and structurally. Motor imagery-based NFB (MI-NFB) can improve motor imagery ability by providing feedback on brain activity during motor imagery, thereby showing effectiveness in performance and motor learning. Furthermore, the effects of MI-NFB are further enhanced when it is combined with noninvasive brain stimulation and motor exercise. Therefore, MI-NFB is used in the physiotherapy of patients with neurological diseases, such as stroke and Parkinson disease, as well as children with attention deficit-hyperactivity disorder and elderly people. This chapter reviews MI-NFB in physiotherapy practice, thus contributing to the development of effective evidence-based physiotherapy.
Keywords
- neurofeedback
- motor imagery
- neurorehabilitation
- stroke
- Parkinson disease
- physiotherapy practice
1. Introduction
Neurofeedback (NFB) is a technique that promotes voluntary control of brain activity by providing feedback on brain activity to the participant [1]. Kamiya found that electroencephalography (EEG) can be used to notice changes in brain states [2] and that it can be controlled spontaneously by feeding back activity in the α-frequency band [3]. These research results were the first step to developing NFB, which has been widely used to treat psychiatric disorders [4], improve cognitive function [5], enhance motor performance [6], and so on. In NFB, brain activity is measured mainly by EEG [7], functional magnetic resonance imaging (fMRI) [8], and functional near-infrared spectroscopy [9], and feedback on the activity in the targeted brain regions is provided to the participant to facilitate self-regulation of the participant’s brain activity. The methods used to provide feedback include visual feedback [10], in which brain activity is projected on a monitor, and auditory feedback [11], which uses sound stimuli of different frequencies. These methods are used to encourage participants to voluntarily adjust their brain activity.
Although many neural mechanisms underlying the effects of NFB are still unclear, several potential mechanisms have been proposed [12, 13, 14], one of which is the induction of neuroplasticity through NFB. In addition to changing motor performance and brain activity, NFB has also been shown to alter the structure of white matter [15]. For these reasons, neuroplasticity, which causes changes in brain structure, has been proposed to be a mechanism underlying the effects of NFB. The second potential mechanism involves changing the connectivity of the brain. The neural network associated with NFB can be divided into control, learning, and forward-processing networks [16]. The control network is composed of the frontoparietal control network, which consists of the dorsolateral frontal and posterior parietal cortices, with the participation of brain regions related to the processing of feedback stimuli, such as the lateral occipital cortex and thalamus. The dorsolateral frontal and posterior parietal cortices are associated with the execution of imagery and other processes [17] and may be important in the mental processes that control brain activity. The learning network consists of the dorsal striatum, which has been shown to be associated with motor control and learning based on procedural memory [18] and may be involved in learning to coordinate neural activity in NFB. Finally, the reward-processing network consists of the anterior insula, anterior cingulate cortex, and ventral striatum. The anterior insula [19] and anterior cingulate cortex [20] are associated with a conscious perception of reward, whereas the ventral striatum is associated with an unconscious perception of reward [21, 22]. The reward-processing network has been reported to be activated by the NFB [23], which is also known to regulate brain activity. Thus, the knowledge of results (KR) for regulating brain activity conferred by the NFB may be involved in the process of recognizing the accomplishment of the task, and these neural networks have been proposed to be related to NFB.
In addition to the NFB-related networks described above, NFB alters the connectivity of the language network and visual network, which are involved in internal reception [24]. NFB has also been reported to alter connectivity not only within the cerebrum but also between the primary motor cortex and the cerebellum [25], and NFB may produce its effects by altering connectivity between these brain regions. As described above, NFB has been suggested to cause functional and structural changes in the brain. This chapter reviews the NFB to motor imagery frequently used in physiotherapy practice.
2. Motor imagery-based neurofeedback
Recently, the effect of NFB on motor imagery has attracted much attention. This approach is called motor imagery-based NFB (MI-NFB). Motor imagery is a cognitive process of imagining what it is like to exercise without actual movement [26]. Motor imagery can be divided into myosensory first-person imagery and visual third-person imagery, and previous studies have reported individual differences in motor imagery ability [27, 28]. Motor imagery is known to improve performance [29] and has a motor-learning effect [30] even when the participant does not actually exercise. In addition, motor imagery has been supported from a neurological aspect, as there are areas where brain activity similar to that of actual exercise is observed [31], and resting EEG is altered by motor imagery [32]. On the basis of these background characteristics, motor imagery has been used in sports training for athletes [33] and in rehabilitation for stroke patients [34, 35]. However, one limitation of motor imagery is that the participant cannot understand the accuracy of the imagery because the actual movement does not occur [36]. NFB can be used to solve this problem. MI-NFB provides real-time feedback on brain activity during motor imagery to the participant and improves the accuracy of imagery by activating brain regions related to motor imagery [37]. In this section, the mechanism and effects of MI-NFB are explained on the basis of the results of studies conducted in healthy individuals.
MI-NFB has been reported to provide feedback on activity in the primary motor cortex (M1) [38, 39] and supplementary motor area (SMA) [40, 41] in many studies. These areas are activated by motor imagery and have been reported to be related to the accuracy of motor imagery [31]. The blood oxygen-level dependent (BOLD) signal in fMRI [40, 41] and the event-related desynchronization (ERD) of EEG β-rhythms [42] and μ-rhythms [43] are mainly used as feedback stimuli for MI-NFB. In this approach, the ERD reflects a decrease in the EEG signal caused by the occurrence of an event [44]. Control of these parameters related to motor imagery is then encouraged to improve the accuracy of motor imagery and motor performance (Figure 1).
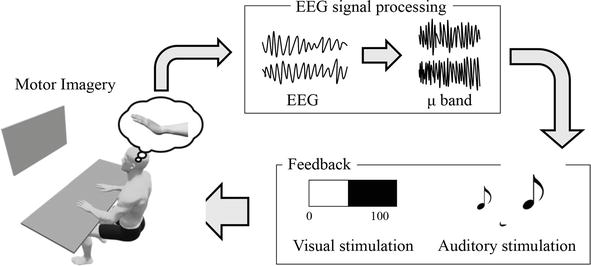
Figure 1.
MI-NFB system using EEG. EEG is measured during motor imagery, and μERD data are used to provide feedback to the patients, including visual feedback using bar graphs or auditory feedback using sound stimuli. This system promotes voluntary control of EEG during motor imagery.
MI-NFB has been proposed to facilitate motor learning by providing KR [45]. KR is one of the basic principles underlying motor learning, and if motor learning involves actual exercise, the results are automatically returned as feedback by behavioral indicators and sensory stimuli, and motor learning is based on this information [46]. In contrast, motor imagery does not provide feedback on KR such as behavioral indices and sensory stimuli because it is not accompanied by actual movement. In this context, MI-NFB provides brain activity as KR, and motor learning is facilitated based on this information.
MI-NFB has been shown to alter brain activity and improve motor imagery ability [37]. Hwang et al. tested the effects of MI-NFB with visual stimuli in healthy young individuals [47]. In their study, right- or left-hand movements were used as the imagery task, the μ activity in the sensorimotor area was displayed on a monitor, and the participants received visual feedback regarding brain activity. The findings showed that this system facilitated control of brain activity during motor imagery. Their results indicated that MI-NFB enabled the control of μ activity, potentially indicating an improvement in motor imagery ability. Additionally, Grigorev et al. examined the effects of MI-NFB with vibrotactile stimulation in healthy young individuals [48]. In their study, the imagery task consisted of right- or left-handed movements. Vibration actuators were attached to the bilateral forearms and behind the neck and set to vibrate when μ-rhythmic ERD occurred, providing vibrotactile brain activity feedback (Figure 2). Their results showed that MI-NFB produced significantly greater ERD for non-dominant hand motor imagery, but it had no significant difference for dominant hand motor imagery. Since non-dominant hand movements require higher brain activity than dominant hand movements [49], the present results may modulate the brain activity mobilized for these non-dominant hand movements. Although MI-NFB has been suggested to enable the control of brain activity and improve the accuracy of motor imagery, as shown in the above study [48], the effect of MI-NFB varies depending on the conditions. Moreover, the effects of MI-NFB are not consistent in relation to the participant’s characteristics [50] and the difficulty of the task [51]. Therefore, improving the accuracy of MI-NFB and evaluating its responsiveness are future research topics.
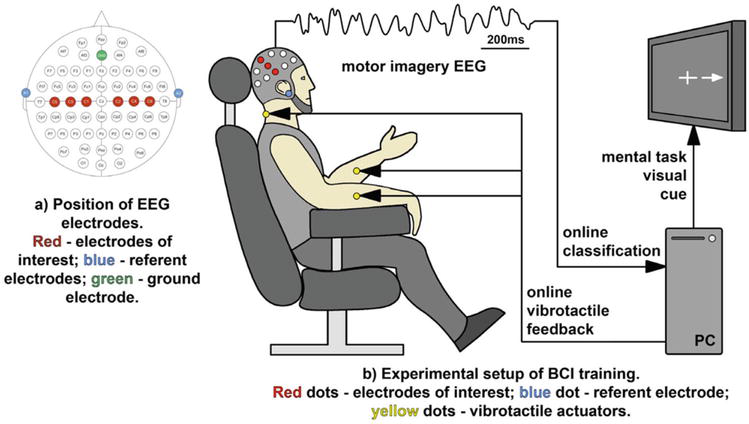
Figure 2.
Experimental setup of MI-NFB using vibrotactile stimulation [
In this section, the basic mechanisms and effects of MI-NFB are described on the basis of previous studies. MI-NFB provides the participant KR in motor imagery accuracy, encouraging the participant to control brain activity related to motor imagery. This process improves the accuracy of motor imagery. Therefore, MI-NFB may be effective in addressing participants’ inability to understand whether their motor imagery is good or poor. However, the effectiveness of MI-NFB varies depending on the difficulty of the task and the participants’ characteristics; hence, more accurate MI-NFB is required in the future.
3. Combination of neurofeedback with other techniques
With the evolving usage of stimulators in physiotherapy [52], it is becoming increasingly clear that MI-NFB should not be used alone; rather, it should be used in combination with some other interventions to further amplify its effects. This section describes the use of MI-NFB in combination with other techniques.
3.1 Combination of neurofeedback with transcranial stimulation
Recent studies have begun examining the efficacy of transcranial stimulation combined with MI-NFB. Transcranial stimulation is classified into transcranial direct current stimulation (tDCS), transcranial alternating current stimulation, and transcranial magnetic stimulation [53]. These stimuli are used to promote brain plasticity and alter cognitive and mental functions [54], and their effects have attracted much attention. The effects of transcranial stimulation on motor imagery have been validated, with tDCS [55, 56], transcranial alternating current stimulation [56, 57], and transcranial magnetic stimulation [58] all shown to improve the accuracy of motor imagery. However, the effects of combining transcranial stimulation with MI-NFB are largely unclear.
In a study examining the effects of tDCS on MI-NFB [59], participants were divided into NFB and NFB + tDCS groups, and MI-NFB for left wrist dorsiflexion was performed. For the NFB + tDCS group, tDCS was performed before imaging. During NFB, EEG measurements were obtained, and the ERD of the μ-rhythm in M1 was projected on the monitor (Figure 3). In tDCS, the anode was placed on C4 and the cathode on the right upper arm, and anodal stimulation was administered to increase the activity of C4, which corresponds to the sensorimotor area of the left hand (Figure 4). The participants performed a motor imagery task without NFB before and after MI-NFB, and the EEG results obtained during the task were compared between the groups. The results showed that the ERD of the μ-rhythm in the NFB + tDCS group was significantly higher than that in the NFB group. This result indicates that the effect of MI-NFB alone, as well as the combination of MI-NFB and tDCS, can amplify the effect of MI-NFB. Both MI-NFB [37] and tDCS [55, 56] have been shown to improve MI accuracy, but the processes underlying their effects are different. Therefore, the effects of MI-NFB and tDCS do not appear to overlap, indicating the possibility of a synergistic effect.

Figure 3.
Experimental setup [
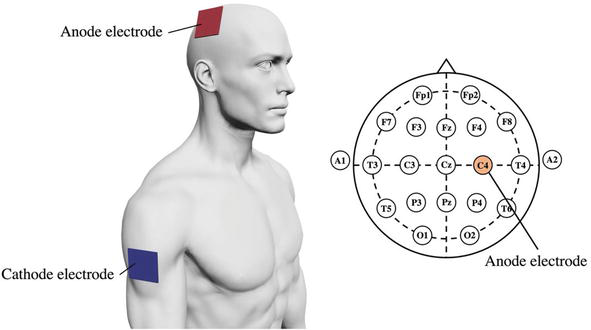
Figure 4.
tDCS setup [
As shown above, the efficacy of MI-NFB combined with tDCS has been demonstrated. Other electrical and magnetic stimuli also have similar mechanisms of action to tDCS, and their use in combination with MI-NFB may amplify the effects of MI-NFB. Intervention methods that combine such techniques are still in their infancy and require further study. In addition, noninvasive brain stimulation methods such as tDCS are not yet commonly used in physiotherapy practice. With advancements in brain science and engineering, physiotherapy using these devices will become widespread and provide effective rehabilitation.
3.2 Combination of neurofeedback with action observation or motor execution
Action observation and motor execution are often compared with motor imagery, and the accuracy of motor imagery and motor performance are improved when action observation and motor execution are combined with motor imagery [60, 61]. The effects of MI-NFB have also been shown to be improved by combining it with action observation and motor execution.
Action observation is a cognitive process of observing a scene in motion without moving [62]. Action observation and motor imagery have both been used in conjunction with motor execution to support motor learning, but both are different techniques, and it was believed that action observation and motor imagery cannot be used together. However, studies elucidating the neural bases and effects of these techniques have clarified that the combination of motor imagery and action observation improves the accuracy of motor imagery and motor performance [63]. Moreover, the effectiveness of the combination of action observation and MI-NFB has been verified in recent studies. Friesen et al. [64] used a handshake task to examine the effects of MI-NFB in combination with action observation. In their study, participants were divided into two groups: an NFB group, which received EEG feedback during the task, and a sham group, which received false stimulus feedback. The participants were required to watch a video of a complex handshake and simultaneously imagine the action (Figure 5). The ERD of μ-rhythms in the sensorimotor area was then measured, and the NFB group received visual feedback of brain activity by changing the saturation of the handshake video. Specifically, a vivid movie was displayed when a sufficient ERD was generated, and a monochrome movie was displayed when the ERD was insufficient (Figure 6). A comparison of ERD between groups showed that the NFB group had significantly higher ERD than the sham group, indicating an improvement in the accuracy of motor imagery when NFB is used in combination with action observation and motor imagery. The combination of motor imagery and action observation has been shown to increase cortical excitability [65] and activate motor-related networks [66]. In contrast, the results of this study showed that the combination of motor imagery and action observation did not have the greatest effect but that the effect was further enhanced when NFB was used in combination.
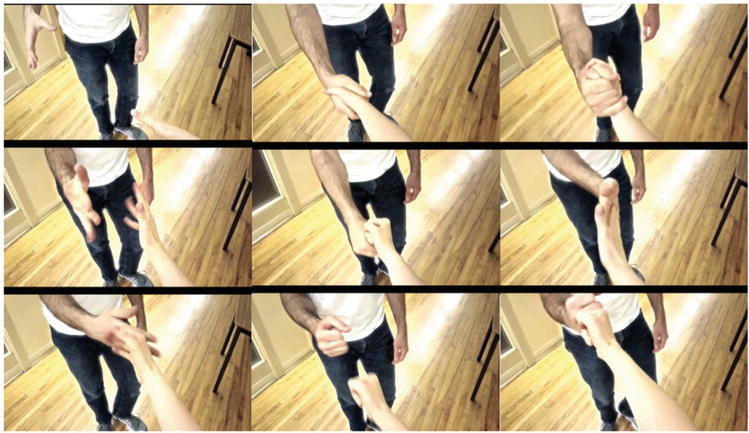
Figure 5.
Action observation of a handshake [
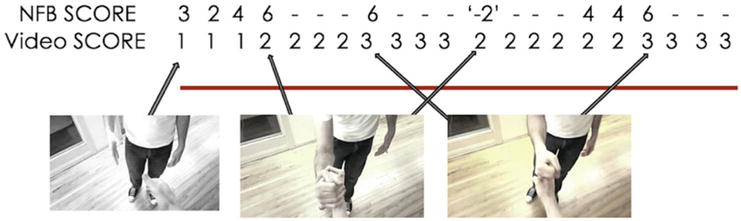
Figure 6.
Providing NFB when action observation and MI-NFB are combined [
Ono et al. [67] examined the combined effects of MI-NFB and action observation using proprioceptive NFB. In their study, participants were divided into an NFB group and a control group in which random feedback was provided, and training was conducted using a combination of action observation and motor imagery. A ball-gripping task was used in this study, and participants imagined the motion while watching a video of themselves gripping a ball. An exoskeleton robot was attached to the hand and set to passively generate finger-flexion movements when ERD with a sufficient μ-rhythm was generated during the task. This task was performed six times at 2-week intervals, and the ERD during motor imagery was continuously evaluated. The results showed a significant increase in the ERD in the NFB group in comparison with the control group. These results indicate that the combination of MI-NFB using proprioceptive and behavioral observations can improve the accuracy of motor imagery. Motor imagery and action observation may have activated motor-related areas, which were further modified by NFB. Similar to the findings of the study by Friesen et al. [64], this study showed that the combination of MI-NFB and action observation was effective. Furthermore, since proprioceptive feedback has been reported to be more effective than visual feedback in MI-NFB [68], the feedback system used in this study may have maximized the effects of motor imagery, action observation, and NFB, respectively. As shown in the above studies, the effectiveness of using MI-NFB in combination with action observation is being verified, and more effective methods of motor imagery training are being developed. Action observation is often used in physiotherapy practice, wherein patients observe the actions of physical therapists. Therefore, the results of these studies can be applied to physiotherapy practice to provide effective rehabilitation.
Motor execution generates myosensory and superficial sensory information that does not occur in motor imagery [69], which is thought to support motor imagery as KR [70]. In addition, motor execution has a neural mechanism that is partially similar to motor imagery [31]. For these reasons, the effects of training with motor execution and motor imagery have been examined [71]. The effects of combining MI-NFB and motor execution have been initially verified in recent studies. In one study, the authors examined the effects of combining MI-NFB and motor execution using a standing postural-control task [72]; the participants in this study were divided into the MIME group, which alternated between motor imagery and motor execution, and the MIME + NFB group, in which NFB was added to the motor imagery at that time. The task consisted of an actual exercise, in which the participant stood on an unstable board and kept the board horizontal, and a phase in which the participant imagined the exercise (Figure 7). The imagery of the exercise was performed in the resting sitting position, and the participants in the MIME + NFB group received ERD feedback of μ-rhythms in the motor-related regions during the imagery. The feedback method was auditory feedback, wherein higher-frequency sound stimuli were provided as the ERD increased. Performance on the standing postural-control task before and after training was compared between groups. The results showed significantly better standing postural control performance in the MIME + NFB group than in the MIME group. These results indicate that the combination of MI-NFB and motor execution improves the accuracy and performance of motor imagery. NFB provided KR of motor imagery from the neural activity aspect, and motor execution provided KR of motor imagery from the superficial and proprioceptive aspects, and their combined use may have improved the accuracy and effectiveness of motor imagery. Thus, the initial studies on the effects of combining MI-NFB and motor execution have suggested that this approach may improve the accuracy of motor imagery. Since there are many situations in physiotherapy practice where actual exercise and motor imagery are used together, the results of these studies can be applied to clinical treatment.
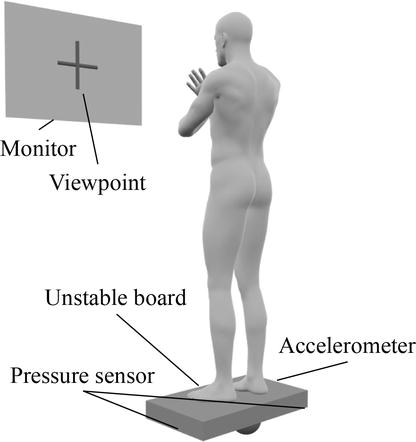
Figure 7.
A standing postural-control task combining MI-NFB and motor execution [
3.3 Summary
This section described the effects of using MI-NFB in combination with other techniques. The use of noninvasive brain stimulation methods that alter brain states similar to NFB as well as the addition of action observation and motor execution, which are comparable to motor imagery, to MI-NFB can improve its effectiveness. However, further research is needed to provide more effective MI-NFB, since many aspects of this technique remain to be verified, including the effects of the area to be stimulated and the intensity of the stimulation. In physiotherapy practice, motor imagery shows individual differences in its effectiveness [36]. Thus, combining it with the other techniques described above could solve this problem and facilitate effective exercise imagery training for all patients, thereby establishing evidence-based motor imagery and MI-NFB.
4. Neurofeedback in physiotherapy practice
MI-NFB has been validated not only in healthy individuals but also in patients with neurological diseases. This section presents clinical studies of MI-NFB in patients with neurological disorders and describes the physiotherapy practice of MI-NFB.
4.1 Neurofeedback for patients with stroke
The hemiplegia and motor impairment caused by stroke necessitate physical therapy [73]. In particular, stroke patients have reduced motor imagery ability [74, 75], and MI-NFB is used to improve the effectiveness of motor imagery training [76]. Moreover, upper limb function is less likely to recover after stroke than lower limb function [77], and MI-NFB has attracted attention as a new and effective intervention method.
Pichiorri et al. [78] examined the effects of MI-NFB on upper limb movement in patients with subacute stroke. In their study, participants were divided into a control group, which performed motor imagery without NFB, and an MI-NFB group, which performed motor imagery with NFB. EEG measurements were obtained during imaging, and the ERD of the β-rhythm was evaluated. In the MI-NFB group, the virtual hand on the monitor moved to provide visual feedback on brain activity. In this study, participants underwent regular rehabilitation, including physical therapy and occupational therapy for 3 hours a day, and motor imagery training was conducted in addition to these sessions. In a comparison of the control and MI-NFB groups, the MI-NFB group showed a significantly higher ERD of the β-rhythm during imagery, and this phenomenon occurred mainly in the impaired hemisphere. This result suggests that MI-NFB may have enhanced the accuracy of motor imagery by facilitating recruitment of the impaired hemisphere during motor imagery. In addition, an intergroup comparison of the functional connectivity of the resting brain after training showed that the MI-NFB group had significantly increased connectivity between hemispheres in high-frequency bands such as β. The details of this phenomenon are not yet clear, but it is suggested to be related to the compensatory functions of the brain. The injured hemisphere and the unaffected hemisphere may be tightly connected, and bilateral activity may have produced the motor imagery. Interhemispheric connectivity was also observed after imagery training, indicating that it may contribute to brain reorganization. In addition, MI-NFB improved motor function as assessed by the arm section of the Fugl-Meyer Assessment (FMA), the upper limb section of the Medical Research Council scale for muscle strength, and the National Institute of Health Stroke Scale. In particular, the FMA score improved by more than a minimal clinically important difference only in the MI-NFB group. These improvements in brain and motor function indicate that MI-NFB is effective in physiotherapy practice for upper limb function in stroke patients. Zich et al. [79] also examined the effects of frequent MI-NFB on home treatment of chronic stroke patients and presented case reports of three chronic stroke patients more than 6 months after stroke onset, one with mild upper limb paralysis and two with severe upper limb paralysis. In MI-NFB, the participants imagined hand movements and received visual feedback of β-rhythm ERDs assessed from EEG. After 4 weeks of home training with MI-NFB, all participants showed cortical activation in motor-related areas. In addition, upper limb motor function improved in participants who showed mild paralysis. This patient clearly showed functional and structural recovery of the central nervous system after the training. This study did not show motor function or structural brain changes in patients with severe paralysis but showed changes in patients with mild paralysis. These findings indicate that MI-NFB may be effective even in patients with chronic stroke. Future studies should continue to validate MI-NFB in chronic stroke patients, allowing for more widespread use of MI-NFB in physiotherapy practice.
MI-NFB has been examined not only for upper limb function but also for gait function. Mihara et al. [41] performed motor imagery training of gait in patients with gait disorders due to subcortical stroke. During the imagery training, frontal functional near-infrared spectroscopy measurements were obtained to provide visual feedback on blood flow in the SMA associated with the motor imagery. The participants were divided into two groups: the MI-NFB group, which received cerebral blood flow feedback, and the sham group, which received sham feedback. The MI-NFB group showed a significant increase in SMA blood flow in comparison with the sham group. In addition to changes in brain function, the MI-NFB group’s score on the Timed Up and Go test, a gait evaluation method, was significantly better after training. The results show that MI-NFB can effectively improve brain and motor function in gait, indicating that MI-NFB can be widely used in physiotherapy practice for stroke patients.
MI-NFB has been shown to improve upper limb and gait functions in stroke patients and may be an effective approach in physiotherapy practice. However, stroke symptoms vary according to the region of injury, and detailed classification of participants and investigation of interventional methods on the basis of the region of injury will contribute to the development of more effective MI-NFB. Conversely, a standard MI-NFB approach for stroke patients could be developed through large-scale studies that are not limited to the region of injury. Further research is needed to establish MI-NFB in physiotherapy practice for stroke patients.
4.2 Neurofeedback for patients with Parkinson disease
Parkinson disease (PD) is a neurological disorder whose main symptoms are resting tremors, muscle stiffness, immobility, and postural dysreflexia [80]. Motor imagery has been shown to be effective in treating these symptoms [81, 82], and MI-NFB is used to improve the effectiveness of motor imagery training. PD patients show decreased motor imagery ability [75, 83], and MI-NFB may contribute to symptom improvement in PD patients.
Subramanian et al. [84] examined the efficacy of MI-NFB in patients with Hoehn and Yahr stage I–III PD. The participants were divided into NFB and control groups and were required to imagine finger-tapping movements of the left hand. fMRI was measured during the imagery, and the BOLD signal of the SMA was returned as feedback to the NFB group. Next, the finger-tapping test and the fMRI BOLD signal during imagery were evaluated before and after training. The finger-tapping test involves tapping the thumb and index finger at maximum speed and reflects the immobility of PD patients [85]. The results showed upregulated SMA brain activity and greater finger-tapping speed in the NFB group in comparison with the control group. The results indicate that MI-NFB modulates brain activity in regions related to PD symptoms and improves immobility. A compensatory network involving the basal ganglia and thalamus is activated in PD as symptoms progress [86]. In addition, SMA projects to the globus pallidus internus of the basal ganglia and thalamus [87, 88], suggesting that MI-NFB may activate the compensatory network. As reported in the above studies, MI-NFB has been shown to be effective in treating motor symptoms in PD patients. Additionally, Subramanian et al. [89] examined the effect of combining exercise therapy and MI-NFB in PD patients. In their study, participants with Hoehn and Yahr stage I–III PD were divided into two groups: the EX group, which received exercise therapy, and the NF group, which received MI-NFB in addition to exercise therapy, and trained for 10 weeks. The EX group performed finger-tapping and postural-control tasks using a virtual reality game device as exercise therapy. In contrast, the NF group performed finger-tapping motor imagery and postural-control tasks. The motor score of the Movement Disorder Society-sponsored revision of the Unified Parkinson’s Disease Rating Scale (MDS-UPDRS) [90] was performed before and after training. The MDS-UPDRS consists of four items: daily motor experiences, daily non-motor experiences, motor tests, and motor complications. In addition, the MDS-UPDRS and other PD symptom scales were assessed before, during, and after the 4-week intervention. The results showed that the motor examination score of MDS-UPDRS significantly improved in the NF group in comparison with the EX group in the unmedicated condition. However, the MDS-UPDRS scores with medication did not show significant group differences for any of the items (Figure 8). This result indicates that MI-NFB may contribute to the recovery of basal ganglia function. In the on-medication condition, PD patients are treated with levodopa and other common PD medications that affect the basal ganglia. However, the findings of the study described above showed a difference in motor function with MI-NFB only in the off-medication condition, suggesting that MI-NFB may be an alternative to pharmacotherapy. Thus, MI-NFB has been shown to influence task performance reflecting motor symptoms as well as a general scale assessing the severity of PD. However, MI-NFB did not improve motor function in the on-medication condition. Since most patients with PD are on medication, the clinical usefulness of MI-NFB requires investigation of approaches to improve motor symptoms while on medication.
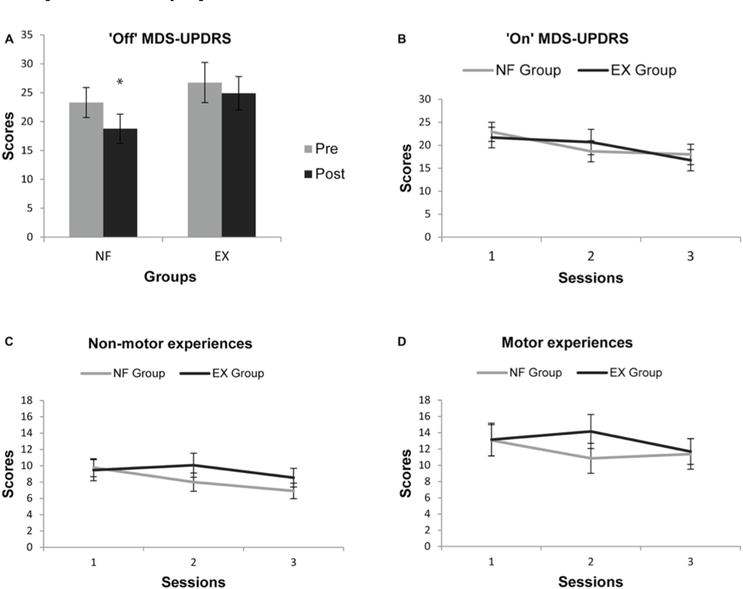
Figure 8.
Effects of MI-NFB combined with exercise therapy in PD patients [
In physiotherapy practice, MI-NFB may be an effective approach to improve motor symptoms such as immobility in PD patients. Since the basal ganglia, which are impaired by PD, are connected to the SMA, which is activated by MI-NFB, MI-NFB can improve symptoms via the SMA. However, the effectiveness of MI-NFB depends on the medication conditions, and further validation is needed to devise an effective MI-NFB protocol. In addition to motor symptoms, PD often presents with non-motor symptoms such as autonomic and psychiatric symptoms. Therefore, the potential effects of non-motor symptoms on the effectiveness of MI-NFB also require consideration.
4.3 Summary
This section presented information regarding the physiotherapy practice of MI-NFB on the basis of its effects on stroke and PD patients; MI-NFB may be effective for stroke and PD caused by brain damage because it encourages the voluntary regulation of brain activity. However, MI-NFB is not always effective for these neurological diseases because their symptoms differ among patients. The findings also indicate the need to develop a method for selecting patients for whom MI-NFB is indicated as well as new MI-NFB protocol that demonstrates a certain level of effectiveness regardless of the characteristics of the patients. With the establishment of additional evidence, MI-NFB may emerge as a standard approach in physiotherapy practice.
5. Conclusion
This chapter described MI-NFB findings from basic studies in healthy individuals and clinical studies in patients with neurological diseases. Many studies have shown that MI-NFB facilitates voluntary control of brain activity and improves motor performance. The use of MI-NFB in combination with other techniques such as transcranial stimulation and action observation has been shown to further enhance its effects. Although clinical studies on patients are still scarce, a certain level of effectiveness has been demonstrated. Therefore, MI-NFB may be an effective approach in the practice of physiotherapy. However, further evidence needs to be developed for the widespread use of MI-NFB; controlling the brain with MI-NFB may raise the level of physiotherapy practice.
Acknowledgments
This work was supported by JSPS KAKENHI (grant number JP23K10417). We would like to thank Editage (www.editage.com) for English language editing.
Appendices and nomenclature
neurofeedback | |
electroencephalography | |
functional magnetic resonance imaging | |
motor imagery-based neurofeedback | |
primary motor cortex | |
supplementary motor area | |
event-related desynchronization | |
knowledge of result | |
transcranial direct current stimulation | |
motor execution | |
Fugl-Meyer assessment | |
Movement Disorder Society-sponsored revision of the Unified Parkinson’s Disease Rating Scale |
References
- 1.
Hampson M, Ruiz S, Ushiba J. Neurofeedback. Neuroimage. 2020; 218 :116473. DOI: 10.1016/j.neuroimage.2019.116473 - 2.
Kamiya J. Operant control of the EEG alpha rhythm and some of its reported effects on consciousness. In: Tart C, editor. Altered States of Consciousness. New York: Wiley; 1969. pp. 507-517 - 3.
Kamiya J. Conscious control of brain waves. Psychology Today. 1968; 1 :57-60 - 4.
Arns M, Batail JM, Bioulac S, Congedo M, Daudet C, Drapier D, et al. Neurofeedback: One of today's techniques in psychiatry? Encephale. 2017; 43 :135-145. DOI: 10.1016/j.encep.2016.11.003 - 5.
Loriette C, Ziane C, Ben HS. Neurofeedback for cognitive enhancement and intervention and brain plasticity. Revue Neurologique (Paris). 2021; 177 :1133-1144. DOI: 10.1016/j.neurol.2021.08.004 - 6.
Onagawa R, Muraoka Y, Hagura N, Takemi M. An investigation of the effectiveness of neurofeedback training on motor performance in healthy adults: A systematic review and meta-analysis. NeuroImage. 2023; 270 :120000. DOI: 10.1016/j.neuroimage.2023.120000 - 7.
Batail JM, Bioulac S, Cabestaing F, Daudet C, Drapier D, Fouillen M, et al. EEG neurofeedback research: A fertile ground for psychiatry? Encephale. 2019; 45 :245-255. DOI: 10.1016/j.encep.2019.02.001 - 8.
Watanabe T, Sasaki Y, Shibata K, Kawato M. Advances in fMRI real-time neurofeedback. Trends in Cognitive Sciences. 2017; 21 :997-1010. DOI: 10.1016/j.tics.2017.09.010 - 9.
Soekadar SR, Kohl SH, Mihara M, von Lühmann A. Optical brain imaging and its application to neurofeedback. NeuroImage: Clinical. 2021; 30 :102577. DOI: 10.1016/j.nicl.2021.102577 - 10.
Shabani F, Nisar S, Philamore H, Matsuno F. Haptic vs. visual neurofeedback for brain training: A proof-of-concept study. IEEE Transactions on Haptics. 2021; 14 :297-302. DOI: 10.1109/TOH.2021.3077492 - 11.
Nakano H, Kodama T, Murata S, Nakamoto T, Fujihara T, Ito Y. Effect of auditory neurofeedback training on upper extremity function and motor imagery ability in a stroke patient: A single case study. International Journal of Clinical Trials. 2018; 3 :126. DOI: 10.15344/2456-8007/2018/126 - 12.
Marzbani H, Marateb HR, Mansourian M. Neurofeedback: A comprehensive review on system design, methodology and clinical applications. Basic and Clinical Neuroscience. 2016; 7 :143-158. DOI: 10.15412/J.BCN.03070208 - 13.
Davelaar EJ. Mechanisms of neurofeedback: A computation-theoretic approach. Neuroscience. 2018; 378 :175-188. DOI: 10.1016/j.neuroscience.2017.05.052 - 14.
Sharon N. Clinical efficacy and potential mechanisms of neurofeedback. Personality & Individual Differences. 2013; 54 :676-686. DOI: 10.1016/j.paid.2012.11.037 - 15.
Sampaio-Baptista C, Neyedli HF, Sanders ZB, Diosi K, Havard D, Huang Y, et al. fMRI neurofeedback in the motor system elicits bidirectional changes in activity and in white matter structure in the adult human brain. Cell Reports. 2021; 37 :109890. DOI: 10.1016/j.celrep.2021.109890 - 16.
Sitaram R, Ros T, Stoeckel L, Haller S, Scharnowski F, Lewis-Peacock J, et al. Closed-loop brain training: The science of neurofeedback. Nature Reviews. Neuroscience. 2017; 18 :86-100. DOI: 10.1038/nrn.2016.164 - 17.
Seeley WW, Menon V, Schatzberg AF, Keller J, Glover GH, Kenna H, et al. Dissociable intrinsic connectivity networks for salience processing and executive control. The Journal of Neuroscience. 2007; 27 :2349-2356. DOI: 10.1523/JNEUROSCI.5587-06.2007 - 18.
Kravitz AV, Matikainen-Ankney BA. Motor control: Memory and motor control in the dorsal striatum. Current Biology. 2020; 30 :R1366-R1368. DOI: 10.1016/j.cub.2020.09.018 - 19.
Billeke P, Ossandon T, Perrone-Bertolotti M, Kahane P, Bastin J, Jerbi K, et al. Human anterior insula encodes performance feedback and relays prediction error to the medial prefrontal cortex. Cerebral Cortex. 2020; 30 :4011-4025. DOI: 10.1093/cercor/bhaa017 - 20.
Umemoto A, HajiHosseini A, Yates ME, Holroyd CB. Reward-based contextual learning supported by anterior cingulate cortex. Cognitive, Affective, & Behavioral Neuroscience. 2017; 17 :642-651. DOI: 10.3758/s13415-017-0502-3 - 21.
Ramot M, Grossman S, Friedman D, Malach R. Covert neurofeedback without awareness shapes cortical network spontaneous connectivity. Proceedings of the National Academy of Sciences of the United States of America. 2016; 113 :E2413-E2420. DOI: 10.1073/pnas.1516857113 - 22.
Daniel R, Pollmann S. A universal role of the ventral striatum in reward-based learning: Evidence from human studies. Neurobiology of Learning and Memory. 2014; 114 :90-100. DOI: 10.1016/j.nlm.2014.05.002 - 23.
Guler S, Cohen AL, Afacan O, Warfield SK. Matched neurofeedback during fMRI differentially activates reward-related circuits in active and sham groups. Journal of Neuroimaging. 2021; 31 :947-955. DOI: 10.1111/jon.12899 - 24.
Dobrushina OR, Vlasova RM, Rumshiskaya AD, Litvinova LD, Mershina EA, Sinitsyn VE, et al. Modulation of intrinsic brain connectivity by implicit electroencephalographic neurofeedback. Frontiers in Human Neuroscience. 2020; 14 :192. DOI: 10.3389/fnhum.2020.00192 - 25.
Vargas P, Sitaram R, Sepúlveda P, Montalba C, Rana M, Torres R, et al. Weighted neurofeedback facilitates greater self-regulation of functional connectivity between the primary motor area and cerebellum. Journal of Neural Engineering. 2021; 18 :056059. DOI: 10.1088/1741-2552/ac2b7e - 26.
Olsson CJ, Nyberg L. Motor imagery: If you can't do it, you won't think it. Scandinavian Journal of Medicine & Science in Sports. 2010; 20 :711-715. DOI: 10.1111/j.1600-0838.2010.01101.x - 27.
Nakano H, Tachibana M, Fujita N, Sawai S, Fujikawa S, Yamamoto R, et al. Reliability and validity of the Japanese Movement Imagery Questionnaire—Revised Second Version. BMC Research Notes. 2022; 15 :334. DOI: 10.1186/s13104-022-06220-y - 28.
Nakano H, Kodama T, Ukai K, Kawahara S, Horikawa S, Murata S. Reliability and validity of the Japanese version of the Kinesthetic and Visual Imagery Questionnaire (KVIQ). Brain Sciences. 2018; 8 :79. DOI: 10.3390/brainsci8050079 - 29.
MacIntyre TE, Madan CR, Moran AP, Collet C, Guillot A. Motor imagery, performance and motor rehabilitation. Progress in Brain Research. 2018; 240 :141-159. DOI: 10.1016/bs.pbr.2018.09.010 - 30.
Ruffino C, Papaxanthis C, Lebon F. Neural plasticity during motor learning with motor imagery practice: Review and perspectives. Neuroscience. 2017; 341 :61-78. DOI: 10.1016/j.neuroscience.2016.11.023 - 31.
Hanakawa T, Dimyan MA, Hallett M. Motor planning, imagery, and execution in the distributed motor network: A time-course study with functional MRI. Cerebral Cortex. 2008; 18 :2775-2788. DOI: 10.1093/cercor/bhn036 - 32.
Sawai S, Fujikawa S, Ushio R, Tamura K, Ohsumi C, Yamamoto R, et al. Repetitive peripheral magnetic stimulation combined with motor imagery changes resting-state EEG activity: A randomized controlled trial. Brain Sciences. 2022; 12 :1548. DOI: 10.3390/brainsci12111548 - 33.
Ridderinkhof KR, Brass M. How kinesthetic motor imagery works: A predictive-processing theory of visualization in sports and motor expertise. Journal of Physiology, Paris. 2015; 109 :53-63. DOI: 10.1016/j.jphysparis.2015.02.003 - 34.
Tong Y, Pendy JT Jr, Li WA, Du H, Zhang T, Geng X, et al. Motor imagery-based rehabilitation: Potential neural correlates and clinical application for functional recovery of motor deficits after stroke. Aging and Disease. 2017; 8 :364-371. DOI: 10.14336/AD.2016.1012 - 35.
Fujikawa S, Ohsumi C, Ushio R, Tamura K, Sawai S, Yamamoto R, et al. Potential applications of motor imagery for improving standing posture balance in rehabilitation. In: Nakano H, editor. Neurorehabilitation and Physical Therapy. London: IntechOpen; 2022. pp. 27-42. DOI: 10.5772/intechopen.105779 - 36.
van der Meulen M, Allali G, Rieger SW, Assal F, Vuilleumier P. The influence of individual motor imagery ability on cerebral recruitment during gait imagery. Human Brain Mapping. 2014; 35 :455-470. DOI: 10.1002/hbm.22192 - 37.
Girges C, Vijiaratnam N, Zrinzo L, Ekanayake J, Foltynie T. Volitional control of brain motor activity and its therapeutic potential. Neuromodulation. 2022; 25 :1187-1196. DOI: 10.1016/j.neurom.2022.01.007 - 38.
Blefari ML, Sulzer J, Hepp-Reymond MC, Kollias S, Gassert R. Improvement in precision grip force control with self-modulation of primary motor cortex during motor imagery. Frontiers in Behavioral Neuroscience. 2015; 9 :18. DOI: 10.3389/fnbeh.2015.00018 - 39.
Berman BD, Horovitz SG, Venkataraman G, Hallett M. Self-modulation of primary motor cortex activity with motor and motor imagery tasks using real-time fMRI-based neurofeedback. NeuroImage. 2012; 59 :917-925. DOI: 10.1016/j.neuroimage.2011.07.035 - 40.
Al-Wasity S, Vogt S, Vuckovic A, Pollick FE. Upregulation of supplementary motor area activation with fMRI neurofeedback during motor imagery. eNeuro. 2021; 8 :ENEURO.0377-18.2020. DOI: 10.1523/ENEURO.0377-18.2020 - 41.
Mihara M, Fujimoto H, Hattori N, Otomune H, Kajiyama Y, Konaka K, et al. Effect of neurofeedback facilitation on poststroke gait and balance recovery: A randomized controlled trial. Neurology. 2021; 96 :e2587-e2598. DOI: 10.1212/WNL.0000000000011989 - 42.
Darvishi S, Gharabaghi A, Boulay CB, Ridding MC, Abbott D, Baumert M. Proprioceptive feedback facilitates motor imagery-related operant learning of sensorimotor β-band modulation. Frontiers in Neuroscience. 2017; 11 :60. DOI: 10.3389/fnins.2017.00060 - 43.
Pineda JA, Silverman DS, Vankov A, Hestenes J. Learning to control brain rhythms: Making a brain-computer interface possible. IEEE Transactions on Neural Systems and Rehabilitation Engineering. 2003; 11 :181-184. DOI: 10.1109/TNSRE.2003.814445 - 44.
Pfurtscheller G, Neuper C. Motor imagery and direct brain-computer communication. Proceedings of the IEEE. 2001; 89 :1123-1134. DOI: 10.1109/5.939829 - 45.
Mihara M, Miyai I, Hattori N, Hatakenaka M, Yagura H, Kawano T, et al. Neurofeedback using real-time near-infrared spectroscopy enhances motor imagery related cortical activation. PLoS One. 2012; 7 :e32234. DOI: 10.1371/journal.pone.0032234 - 46.
Winstein CJ. Knowledge of results and motor learning—Implications for physical therapy. Physical Therapy. 1991; 71 :140-149. DOI: 10.1093/ptj/71.2.140 - 47.
Hwang HJ, Kwon K, Im CH. Neurofeedback-based motor imagery training for brain-computer interface (BCI). Journal of Neuroscience Methods. 2009; 179 :150-156. DOI: 10.1016/j.jneumeth.2009.01.015 - 48.
Grigorev NA, Savosenkov AO, Lukoyanov MV, Udoratina A, Shusharina NN, Kaplan AY, et al. A BCI-based vibrotactile neurofeedback training improves motor cortical excitability during motor imagery. IEEE Transactions on Neural Systems and Rehabilitation Engineering. 2021; 29 :1583-1592. DOI: 10.1109/TNSRE.2021.3102304 - 49.
Boe S, Gionfriddo A, Kraeutner S, Tremblay A, Little G, Bardouille T. Laterality of brain activity during motor imagery is modulated by the provision of source level neurofeedback. NeuroImage. 2014; 101 :159-167. DOI: 10.1016/j.neuroimage.2014.06.066 - 50.
Daeglau M, Zich C, Kranczioch C. The impact of context on EEG motor imagery neurofeedback and related motor domains. Current Behavioral Neuroscience Reports. 2021; 8 :90-101. DOI: 10.1007/s40473-021-00233-w - 51.
Bauer R, Vukelić M, Gharabaghi A. What is the optimal task difficulty for reinforcement learning of brain self-regulation? Clinical Neurophysiology. 2016; 127 :3033-3041. DOI: 10.1016/j.clinph.2016.06.016 - 52.
Ushio R, Tamura K, Fujikawa S, Ohsumi C, Sawai S, Yamamoto R, et al. Clinical application of repetitive peripheral magnetic stimulation in rehabilitation. In: Nakano H, editor. Neurorehabilitation and Physical Therapy. London: IntechOpen; 2022. pp. 43-54. DOI: 10.5772/intechopen.105787 - 53.
Antal A, Luber B, Brem AK, Bikson M, Brunoni AR, Cohen Kadosh R, et al. Non-invasive brain stimulation and neuroenhancement. Clinical Neurophysiology Practice. 2022; 7 :146-165. DOI: 10.1016/j.cnp.2022.05.002 - 54.
Yavari F, Jamil A, Mosayebi Samani M, Vidor LP, Nitsche MA. Basic and functional effects of transcranial electrical stimulation (tES)—An introduction. Neuroscience and Biobehavioral Reviews. 2018; 85 :81-92. DOI: 10.1016/j.neubiorev.2017.06.015 - 55.
Saruco E, Di Rienzo F, Nunez-Nagy S, Rubio-Gonzalez MA, Jackson PL, Collet C, et al. Anodal tDCS over the primary motor cortex improves motor imagery benefits on postural control: A pilot study. Scientific Reports. 2017; 7 :480. DOI: 10.1038/s41598-017-00509-w - 56.
Xie J, Peng M, Lu J, Xiao C, Zong X, Wang M, et al. Enhancement of event-related desynchronization in motor imagery based on transcranial electrical stimulation. Frontiers in Human Neuroscience. 2021; 15 :635351. DOI: 10.3389/fnhum.2021.635351 - 57.
Feurra M, Pasqualetti P, Bianco G, Santarnecchi E, Rossi A, Rossi S. State-dependent effects of transcranial oscillatory currents on the motor system: What you think matters. The Journal of Neuroscience. 2013; 33 :17483-17489. DOI: 10.1523/JNEUROSCI.1414-13.2013 - 58.
Shu X, Chen S, Chai G, Sheng X, Jia J, Zhu X. Neural modulation by repetitive transcranial magnetic stimulation (rTMS) for BCI enhancement in stroke patients. In: 40th Annual International Conference of the IEEE Engineering in Medicine and Biology Society (EMBC); 18-21 July 2018; Honolulu, HI: IEEE; 2018. pp. 2272-2275. DOI: 10.1109/embc.2018.8512860 - 59.
Sawai S, Murata S, Fujikawa S, Yamamoto R, Shima K, Nakano H. Effects of neurofeedback training combined with transcranial direct current stimulation on motor imagery: A randomized controlled trial. Frontiers in Neuroscience. 2023; 17 :1148336. DOI: 10.3389/fnins.2023.1148336 - 60.
McCormick SA, Causer J, Holmes PS. Active vision during action execution, observation and imagery: Evidence for shared motor representations. PLoS One. 2013; 8 :e67761. DOI: 10.1371/journal.pone.0067761 - 61.
Hardwick RM, Caspers S, Eickhoff SB, Swinnen SP. Neural correlates of action: Comparing meta-analyses of imagery, observation, and execution. Neuroscience and Biobehavioral Reviews. 2018; 94 :31-44. DOI: 10.1016/j.neubiorev.2018.08.003 - 62.
Jeannerod M. Neural simulation of action: A unifying mechanism for motor cognition. NeuroImage. 2001; 14 :S103-S109. DOI: 10.1006/nimg.2001.0832 - 63.
Vogt S, Di Rienzo F, Collet C, Collins A, Guillot A. Multiple roles of motor imagery during action observation. Frontiers in Human Neuroscience. 2013; 7 :807. DOI: 10.3389/fnhum.2013.00807 - 64.
Friesen CL, Bardouille T, Neyedli HF, Boe SG. Combined action observation and motor imagery neurofeedback for modulation of brain activity. Frontiers in Human Neuroscience. 2017; 10 :692. DOI: 10.3389/fnhum.2016.00692 - 65.
Chye S, Valappil AC, Wright DJ, Frank C, Shearer DA, Tyler CJ, et al. The effects of combined action observation and motor imagery on corticospinal excitability and movement outcomes: Two meta-analyses. Neuroscience and Biobehavioral Reviews. 2022; 143 :104911. DOI: 10.1016/j.neubiorev.2022.104911 - 66.
Nedelko V, Hassa T, Hamzei F, Schoenfeld MA, Dettmers C. Action imagery combined with action observation activates more corticomotor regions than action observation alone. Journal of Neurologic Physical Therapy. 2012; 36 :182-188. DOI: 10.1097/NPT.0b013e318272cad1 - 67.
Ono Y, Wada K, Kurata M, Seki N. Enhancement of motor-imagery ability via combined action observation and motor-imagery training with proprioceptive neurofeedback. Neuropsychologia. 2018; 114 :134-142. DOI: 10.1016/j.neuropsychologia.2018.04.016 - 68.
Vukelić M, Gharabaghi A. Oscillatory entrainment of the motor cortical network during motor imagery is modulated by the feedback modality. NeuroImage. 2015; 111 :1-11. DOI: 10.1016/j.neuroimage.2015.01.058 - 69.
Savaki HE, Raos V. Action perception and motor imagery: Mental practice of action. Progress in Neurobiology. 2019; 175 :107-125. DOI: 10.1016/j.pneurobio.2019.01.007 - 70.
Guillot A, Di Rienzo F, Macintyre T, Moran A, Collet C. Imagining is not doing but involves specific motor commands: A review of experimental data related to motor inhibition. Frontiers in Human Neuroscience. 2012; 6 :247. DOI: 10.3389/fnhum.2012.00247 - 71.
Wriessnegger SC, Steyrl D, Koschutnig K, Müller-Putz GR. Short time sports exercise boosts motor imagery patterns: Implications of mental practice in rehabilitation programs. Frontiers in Human Neuroscience. 2014; 8 :469. DOI: 10.3389/fnhum.2014.00469 - 72.
Sawai S, Fujikawa S, Ohsumi C, Ushio R, Tamura K, Yamamoto R, et al. Effects of neurofeedback on standing postural control task with combined imagined and executed movements. Frontiers in Neuroscience. 2023; 17 :1199398. DOI: 10.3389/fnins.2023.1199398 - 73.
Winstein CJ, Stein J, Arena R, Bates B, Cherney LR, Cramer SC, et al. Guidelines for adult stroke rehabilitation and recovery: A guideline for healthcare professionals from the American Heart Association/American Stroke Association. Stroke. 2016; 47 :e98-e169. DOI: 10.1161/STR.0000000000000098 - 74.
Braun N, Kranczioch C, Liepert J, Dettmers C, Zich C, Büsching I, et al. Motor imagery impairment in postacute stroke patients. Neural Plasticity. 2017; 2017 :4653256. DOI: 10.1155/2017/4653256 - 75.
McInnes K, Friesen C, Boe S. Specific brain lesions impair explicit motor imagery ability: A systematic review of the evidence. Archives of Physical Medicine and Rehabilitation. 2016; 97 :478-489.e1. DOI: 10.1016/j.apmr.2015.07.012 - 76.
Dettmers C, Braun N, Büsching I, Hassa T, Debener S, Liepert J. Neurofeedback-based motor imagery training for rehabilitation after stroke. Der Nervenarzt. 2016; 87 :1074-1081. DOI: 10.1007/s00115-016-0185-y - 77.
Liu M, Fujiwara T, Shindo K, Kasashima Y, Otaka Y, Tsuji T, et al. Newer challenges to restore hemiparetic upper extremity after stroke: HANDS therapy and BMI neurorehabilitation. Hong Kong Physiotherapy Journal. 2012; 30 :83-92. DOI: 10.1016/j.hkpj.2012.05.001 - 78.
Pichiorri F, Morone G, Petti M, Toppi J, Pisotta I, Molinari M, et al. Brain-computer interface boosts motor imagery practice during stroke recovery. Annals of Neurology. 2015; 77 :851-865. DOI: 10.1002/ana.24390 - 79.
Zich C, Debener S, Schweinitz C, Sterr A, Meekes J, Kranczioch C. High-intensity chronic stroke motor imagery neurofeedback training at home: Three case reports. Clinical EEG and Neuroscience. 2017; 48 :403-412. DOI: 10.1177/1550059417717398 - 80.
Tysnes OB, Storstein A. Epidemiology of Parkinson's disease. Journal of Neural Transmission (Vienna). 2017; 124 :901-905. DOI: 10.1007/s00702-017-1686-y - 81.
Cuomo G, Maglianella V, Ghanbari Ghooshchy S, Zoccolotti P, Martelli M, Paolucci S, et al. Motor imagery and gait control in Parkinson's disease: Techniques and new perspectives in neurorehabilitation. Expert Review of Neurotherapeutics. 2022; 22 :43-51. DOI: 10.1080/14737175.2022.2018301 - 82.
Nascimento IAPDS, Santiago LMM, de Souza AA, Pegado CL, Ribeiro TS, Lindquist ARR. Effects of motor imagery training of Parkinson's disease: A protocol for a randomized clinical trial. Trials. 2019; 20 :626. DOI: 10.1186/s13063-019-3694-8 - 83.
Heremans E, Feys P, Nieuwboer A, Vercruysse S, Vandenberghe W, Sharma N, et al. Motor imagery ability in patients with early- and mid-stage Parkinson disease. Neurorehabilitation and Neural Repair. 2011; 25 :168-177. DOI: 10.1177/1545968310370750 - 84.
Subramanian L, Hindle JV, Johnston S, Roberts MV, Husain M, Goebel R, et al. Real-time functional magnetic resonance imaging neurofeedback for treatment of Parkinson's disease. The Journal of Neuroscience. 2011; 31 :16309-16317. DOI: 10.1523/JNEUROSCI.3498-11.2011 - 85.
Picillo M, Vincos GB, Kern DS, Fox SH, Lang AE, Fasano A. Learning more from finger tapping in Parkinson's disease: Up and down from dyskinesia to bradykinesia. Movement Disorders Clinical Practice. 2015; 3 :184-187. DOI: 10.1002/mdc3.12246 - 86.
Park DC, Reuter-Lorenz P. The adaptive brain: Aging and neurocognitive scaffolding. Annual Review of Psychology. 2009; 60 :173-196. DOI: 10.1146/annurev.psych.59.103006.093656 - 87.
Ma L, Wang B, Narayana S, Hazeltine E, Chen X, Robin DA, et al. Changes in regional activity are accompanied with changes in inter-regional connectivity during 4 weeks motor learning. Brain Research. 2010; 1318 :64-76. DOI: 10.1016/j.brainres.2009.12.073 - 88.
Nambu A, Takada M, Inase M, Tokuno H. Dual somatotopical representations in the primate subthalamic nucleus: Evidence for ordered but reversed body-map transformations from the primary motor cortex and the supplementary motor area. The Journal of Neuroscience. 1996; 16 :2671-2683. DOI: 10.1523/JNEUROSCI.16-08-02671.1996 - 89.
Subramanian L, Morris MB, Brosnan M, Turner DL, Morris HR, Linden DE. Functional magnetic resonance imaging neurofeedback-guided motor imagery training and motor training for Parkinson's disease: Randomized trial. Frontiers in Behavioral Neuroscience. 2016; 10 :111. DOI: 10.3389/fnbeh.2016.00111 - 90.
Goetz CG, Tilley BC, Shaftman SR, Stebbins GT, Fahn S, Martinez-Martin P, et al. Movement Disorder Society-sponsored revision of the Unified Parkinson's Disease Rating Scale (MDS-UPDRS): Scale presentation and clinimetric testing results. Movement Disorders. 2008; 23 :2129-2170. DOI: 10.1002/mds.22340