Abstract
Equilibrium molecular dynamics (EMD) simulation has been used to investigate structural behaviors (order-disorder structures) of three-dimensional (3D) strongly coupled dusty plasmas (SCDPs). The Yukawa (screened coulomb) potential and periodic boundary conditions (PBCs) have been used in the SCDPs algorithm. Two factors have been used to analyze the structural behavior of SCDP which are radial distribution function (RDF), and lattice correlation (LC). The results for these factors have been calculated in a canonical (NVT) ensemble at external electric field strength (E* = 0.03) for different plasma conditions of Coulomb coupling (Γ) and Debye screening parameters (κ) at the number of particles (N = 500). Their results have shown that the 3D SCDP structure moves from a disordered to an ordered state with increasing Γ, and the long-range order moves to high Γ with an increase of κ. In comparison to earlier numerical, experimental, and theoretical data, the obtained results have been found to be more acceptable.
Keywords
- lattice correlation
- radial distribution function
- dusty plasma
- molecular dynamics
- electric field strength
1. Introduction
Now, complex liquids have become a matter of interest for many researchers. Different techniques such as experimental, theoretical, and simulation are used to study the behavior of complex liquids. Unambiguous models have been used to describe physical properties for a specific range of temperature and pressure. Explicit equations can also be used to calculate the thermophysical properties of liquids when there is not any literature on complex liquids present. The thermophysical properties of complex liquids are altered with the change in temperature, pressure, and material composition, but the chemical properties stay unaffected. The complex liquids’ phase change is described by thermophysical properties. These are divided into two categories transport and thermodynamic properties. Thermodynamic properties explain the system equilibrium conditions which comprise of entropy, heat capacity, internal energy, pressure, density, and enthalpy while the transport properties contain thermal conductivity, viscosity, RDF, waves with its instabilities, and diffusion. These transport properties state the momentum and energy transfer to the system under concern. These properties help in system designing and have knowledge of the physical mechanism. These properties have many applications in industry and laboratory [1].
1.1 Plasma
About 99% of matter that exists in space is plasma and it is called the fourth state of matter. Mainly, plasma occurs in electrified gas form, where atoms are dissociated into positive ions and electrons. It is a form of matter in different areas of Physics such as technical plasma, terrestrial plasma, solid-state plasma, space plasma, and astrophysics. Plasma is produced artificially in laboratories used for numerous applications likely in a display, fluorescent lights, fusion energy research, and others. The term “plasma” was first time used by Irving Langmuir, who is an American physicist and he defined plasma as “plasma is a quasineutral gas of charged and neutral particles which shows collective behavior”. The neutral gas ionization contains equal numbers of positive and negative charge carriers (ni ≈ ne ≈ n). Where ni is ion density, ne is electron density, and n is number density. Such plasma is called “quasi-neutral”. Collective behavior means that plasma shows a simultaneous response of many particles to an external stimulus. The Debye spheres that surround small particles in plasma are distorted, which allow an externally applied electric field to regulate the interparticle interaction [2]. The main examples of plasma are planetary objects, nuclear fireballs, neon signs, plasma balls, and welding arcs. Plasma is widely used in the field of science and technology. It has a very important part in our daily life. It is used in gas lasers, sterilizing of medical instruments, industries, gas discharge, intense power beams, lightning, water purification plant, and many more [3].
1.2 Plasma history
Irving Langmuir American scientist defined plasma for the first time in 1922. Some academics also worked on plasma physics in 1930. Hans Alfven developed hydromagnetic waves in 1940; these waves are known as Alfven waves, and they were used to explore astrophysical plasma. Beginning around the same time in the Soviet Union, Britain, and the United States in 1950, magnetic fusion energy research was initiated. The study of magnetic fusion energy was regarded as a subfield of thermonuclear power in 1958. By using a Russian Tokomak design, plasma with various plasma properties was produced at the end of 1960. Numerous sophisticated tokamaks that were created between 1970 and 1980 validated the effectiveness of tokamaks. Additionally, the tokamak nearly achieved a fusion break in 1990, and the research on DP physics had begun. DP is defined as “when charged particles absorbed in plasma, becomes four components plasma containing ions, electrons, neutral and dust particles” and dust particles change the plasma properties which is called “dusty plasma” [4].
1.3 Types of plasma
The plasma can be categorized on the bases of the Coulomb coupling factor (Γ) into two categories.
1.3.1 Ideal plasma (weakly coupled plasma)
A plasma is called ideal plasma if Γ ∼
1.3.2 Nonideal plasma (strongly coupled plasma)
A plasma is called nonideal plasma if Γ =
1.4 Complex (dusty) plasmas (CDPs)
DP is a complex many-component plasmas containing ions, electrons, neutral particles, and dust particles. Some plasmas naturally contain dust particles or they may be introduced artificially through different mechanisms e.g., sputtering, etching, etc. CDPs contain dust particles of sizes ranging from tens of nanometers to hundreds of microns. The size of the particle is 3e−8g. DP properties become more complex when charged particles are absorbed in plasma and also when used on the laboratory scale. DP is called as CDP in similarity to the condensed matter field of “complex liquids” in soft matter (polymers, colloidal suspensions, surfactants, etc.). Studies of solitons, shock waves, crystallization and melting fronts, and crystal dislocations are just a few examples of the experiments that have been inspired by this concept. CDP, which can be utilized for similar reasons but differ from colloidal suspensions in having weak damping, make it possible to investigate various processes on their inherent dynamic time scale [6]. Dust particles can be in solid (metallic), liquid, gaseous, crystal, or dielectric form. On the basis of the ordering of many radii and characteristic lengths between particles interacting (rd, λD), plasma with dust particles can be called either “dusty plasma” or “dust in plasma”. If the λD > rd then it is called “dusty plasma” and if λD < rd then it is called “dust in plasma”. Here λD is dust particles Debye length and rd is the interparticle distance [4, 7].
1.5 History of dusty plasma
In 1924, the term plasma was first time defined by Irving Langmuir. In 1980, a very exciting incident happened in the field of DP for the Saturn ring and in laboratory measure of DP dynamical structure parameter was performed. Hannes Alfven and Lyman Spitzer proved dust particles as an important component and images from the Saturn ring have shown that dust particles are rotating around the Saturn ring, having the shape of spokes. The Ulysses European spacecraft passed by Jupiter in 1992, detecting dust particles and measuring their weights and collision speeds. In 1995, NASA’s Galileo spacecraft detected the source of streams of dust around Jupiter. In 1997, Mendis discovered a bright comet by a distant ancestor. The distant ancestor is an extraordinary comic laboratory for the investigation and study of interactions between dust particles and their dynamic and physical behaviors. Other displays of DP were noctilucent clouds, origin nebula, zodiac light, etc. The research from the last analysis has shown that these dust particles are fine particles. The present condition (2000–2017) of DP is stable and is playing a very dominant role in science, industries, technology, energy sectors, and medical stores [8].
1.6 Charge on dust particle
Dust particles have almost e4 order of charge. Their charge is mostly negative in low-temperature laboratory plasma but depending on the charging process charge may be positive. Charging of dust particles can be drained through different processes involving the background plasma (electron and ion) being bombarded on the surface of dust particles, secondary electron production, ion sputtering, photoelectronic emission by UV radiations, etc. When an electron from the background plasma strikes the surface of a dust particle, it gets an electric charge. As electrons are more mobile than ions, the surface dust particle collects electrons, attracts ions, and repels electrons until the state of immobility is attained. Other collective phenomena and wave instabilities are created due to interactions between these particles. This charge is accountable for a long lifetime of particles and confinement in plasma. Dust particle potential is reliant on some parameters such as grain composition, grain size, plasma condition, temperature, and velocity [7].
1.7 Formation of dusty plasma in laboratory
In-lab manufacturing of DP has been expanded using a variety of techniques. Various techniques, including modified Q machines, DC discharges, and RF discharges, have been applied recently. The modified Q machine, a single-ended device used to produce DP, permits the dust particles to disperse throughout a portion of the cylindrical plasma column. Dust particles smaller than a micron form DP in the stratum of dc neon glow discharge. Cold electrodes in a glass tube with a cylinder shape are used to create the discharge. The electrodes are 40 cm apart. Neon pressure varies from 0.2 to 1 Torr, while discharge current varies from 0.4 to 2.5 mA. In a symmetric cylindrical rf plasma system, DP is restrained. The grounded electrodes, the hollow outer electrode, capacitively connected to a 14 MHz rf power amplifier, and the glass window make up the system of RF discharges. Thermonuclear fireballs, dust precipitators, rocket exhaust, dust in space stations, and laboratory-generated DP are examples of man-made DP. Microelectronics fabrication, Plasma Enhanced Chemical Vapor Deposition, flat-panel displays, solar cells, and semiconductor chips are among further uses for DP. DP devices (DPDs) are used in laboratories to create DP. Ordinary flames, candle flames, intense desire, fire, and blaze created by burning gas are essentially weakly ionized plasma with dust particles. The degree of ionization in typical hydrocarbon burns is increased by the thermionic electron emission of 10 nm dust particles. DP also contains in-charged snow and volcanos [9, 10].
2. Methodology
To evaluate the interactions and movements of atoms and molecules at a given time period and to investigate the time relating role of the molecular system a computational method MD simulation is used. It is reliant on Newton’s second law of motion, stated as F = ma. The
In the above equation,
However, particle interaction occurs under the effect of
where z indicates unit vector direction along the z-axis and
System particle is expressed by plasma parameters. Coulomb coupling strength (Γ) is defined as the potential energy of interaction between particles and the average kinetic energy of the particles. The Γ defines the distribution of plasma. Γ is given in this form:
In Eq. (2),
In this velocity verlet algorithm is used to allot initial values of acceleration and velocity to the atom. It is a 3D Ewald summation method for calculation of RDF, and LC. This method is used for long-range interactions in periodic systems [13]. In this section, the EMD simulation is described for RDF, and LC of SCDP for an extensive range of plasma parameters of (1
The lack of any permanent structure characterizes the fluid condition. Nonetheless, there are well-structural correlations that may be studied experimentally to reveal essential insights regarding molecular structure. For systems that are spatially homogeneous, simply relative separation is significant, causing a sum over atom pairs,
and it is possible to average the function over angles without information loss. The
LC gives an idea of structural behavior (order-disorder (OD) structure). In this, we used the EMD technique for the simulation of long-term order and Yukawa potential. In this, we will find density at a point
Long-term crystalline order will be calculated using Fourier transform
The 3D Yukawa liquids (DP) motion is simulated by means of the above equation and SCCDPs OD structures can be found, and in this equation k denotes the OD position reciprocal lattice vector (RLV) of the existing Yukawa system. Here k is given as k = 2π/(1,-1,1)λ, when the structure is fcc then k is RLV and has been used in our EMD numerical code, where edge length is denoted by λ and
3. EMD simulation results
3.1 Lattice correlation as a function of time Ψ(t )
3.1.1 Radial distribution function as a function of distance g(r)
Computational results using EMD simulation have shown in Figure 1 for SCDP LC, Ψ(t) as a function of time at
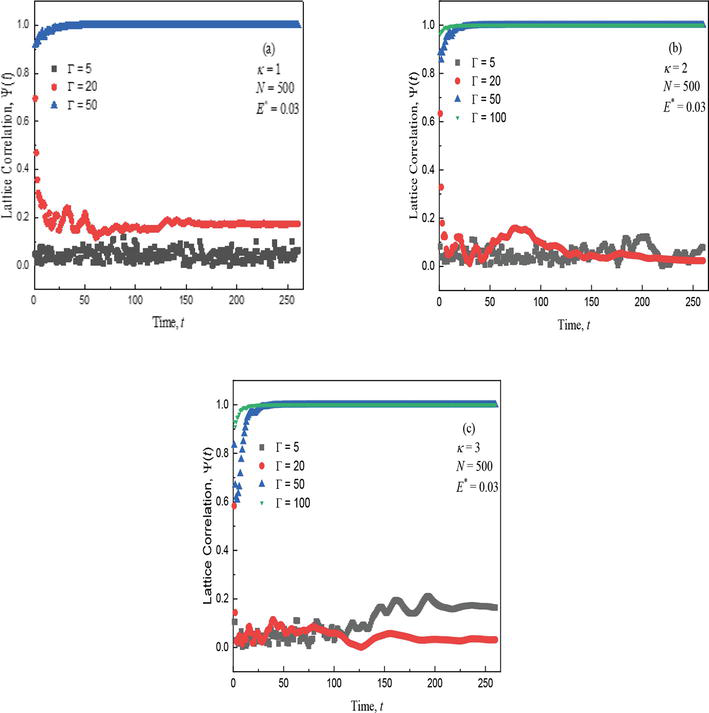
Figure 1.
The variation of LC, Ψ(t) as a function of time of SCDP at system size N = 500, E* = 0.03, and Γ = 5, 20, 50, and 100 for three different plasma factors (a) κ = 1, (b) κ = 2, and (c) κ = 3.
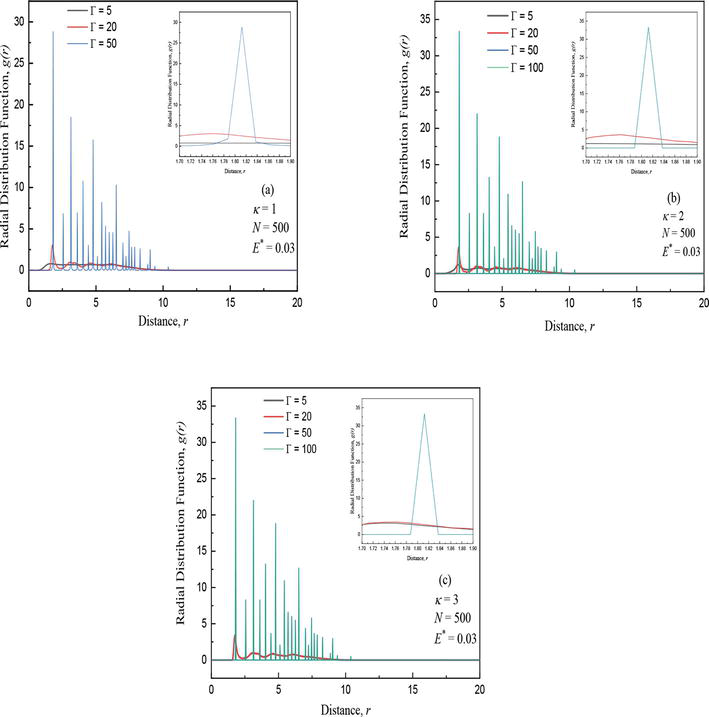
Figure 2.
The variation of RDF, g(r) as a function of distance of SCDP at system size N = 500, E* = 0.03, and Γ = 5, 20, 50, and 100 for three different plasma factors (a) κ = 1, (b) κ = 2, and (c) κ = 3.
4. Conclusions
Two factors LC and RDF have been used for the structural analysis of
Abbreviations
Dusty plasma | |
Complex dusty plasma | |
Equilibrium molecular dynamics | |
Coulomb coupling | |
Debye screening strength | |
Molecular dynamics | |
Periodic boundary conditions | |
Strongly coupled dusty plasmas | |
Weakly coupled plasma | |
External electric field | |
Strongly coupled plasma | |
Lattice correlation | |
Radial distribution function | |
Periodic boundary conditions | |
Three-dimensional | |
Canonical ensemble | |
Number of particles | |
Kinetic energy | |
Potential energy | |
Face-centered cubic | |
Order-disorder | |
Reciprocal lattice vector |
References
- 1.
Shahzad A, He MG. Thermal conductivity calculation of complex (dusty) plasmas. Physics of Plasmas. 2012; 19 (8):083707 - 2.
Ivlev AV, Brandt PC, Morfill GE, Rath C, Thomas HM, Joyce G, et al. Electrorheological complex plasmas. IEEE Transactions on Plasma Science. 2010; 38 (4):733-740 - 3.
Chen FF. Introduction to Plasma Physics and Controlled Fusion. Vol. 1. New York: Plenum press; 1984. pp. 19-51 - 4.
Bellan PM. Fundamentals of Plasma Physics. New York, US: Cambridge University Press; 2008 - 5.
Shahzad A, He MG. Structural order and disorder in strongly coupled Yukawa liquids. Physics of Plasmas. 2016; 23 (9):093708 - 6.
Kompaneets R, Morfill GE, Ivlev AV. Interparticle attraction in 2D complex. Physical Review Letters. 2016; 116 (12):125001 - 7.
Rodriguez IJ. Some Assembly Required: Computational Simulations of Dusty Plasma [University Honors Theses]. Portland, US: Portland State University; 2018. p. 622. DOI: 10.15760/honors.622 - 8.
Shahzad A, Shakoori MA, Mao-Gang HE, Feng Y. Numerical approach to dynamical structure factor of dusty plasmas. In: Plasma Science and Technology-Basic Fundamentals and Modern Applications. Books on Demand. Croatia/London, UK: INTECH Publisher; 2019 - 9.
Shukla PK, Mamun AA. Introduction to Dusty Plasma Physics. Florida, US: CRC Press; 2015 - 10.
Shahzad A, Khan MQ, Shakoori MA, He M, Feng Y. Thermal conductivity of dusty plasmas through molecular dynamics simulations. In: Thermophysical Properties of Complex Materials. Vol. 13. BoD – Books on Demand. Croatia/London, UK: INTECH Publisher; 2020 - 11.
Reshetniak VV, Filippov AV. Properties of yukawa crystals and liquid under phase equilibrium conditions. Journal of Experimental and Theoretical Physics. 2019; 129 :459-469 - 12.
Shakoori MA, He M, Shahzad A. Diffusion coefficients of dusty plasmas in electric field. The European Physical Journal D. 2022; 76 (11):227 - 13.
Shahzad A, He MG, He K. Diffusion motion of two-dimensional weakly coupled complex (dusty) plasmas. Physica Scripta. 2013; 87 (3):035501 - 14.
Rapaport DC. The Art of Molecular Dynamics Simulation. New York, US: Cambridge University Press; 2004 - 15.
Maity S, Das A, Kumar S, Tiwari SK. Interplay of single particle and collective response in molecular dynamics simulation of dusty plasma system. Physics of Plasmas. 2018; 25 (4):043705 - 16.
Evans DJ. Homogeneous NEMD algorithm for thermal conductivity—Application of non-canonical linear response theory. Physics Letters A. 1982; 91 (9):457-460 - 17.
Khrapak SA, Klumov BA, Khrapak AG. Collective modes in two-dimensional one-component-plasma with logarithmic interaction. Physics of Plasmas. 2016; 23 (5):052115 - 18.
Donkó I, Hartmann P, Donkó Z. Molecular dynamics simulation of a two-dimensional dusty plasma. American Journal of Physics. 2019; 87 (12):986-993 - 19.
Shahzad A, Kashif M, Munir T, Perveen A, He M, Bashir S. Calculations of uniaxial tensile strength of Al–Cu–Ni based metallic glasses using molecular dynamics simulations. Physica B: Condensed Matter. 2021; 602 :412566 - 20.
Sukhinin GI, Fedoseev AV, Salnikov MV, Rostom A, Vasiliev MM, Petrov OF. Plasma anisotropy around a dust particle placed in an external electric field. Physical Review E. 2017; 95 (6):063207 - 21.
Kittner M, Klapp SH. Screening effects on structure and diffusion in confined charged colloids. The Journal of Chemical Physics. 2007; 126 (15):154902