Abstract
Advanced ceramic coatings have been largely used in several industrial fields such as aerospace, automotive, power generation, medical or petrochemical, in order to protect or functionalise the surface of different materials. In modern industries, thermal spray processes are the most used ones to manufacture advanced ceramic coatings due to their cost advantages, flexibility and efficiency in processing ceramic materials, especially those with high melting temperature. This chapter provides a brief overview of the progress and current state of different thermal sprayed ceramics and summarises the future trend in this field. Therefore, various advanced ceramics, such as yttria-stabilised zirconia, alumina, hydroxyapatite and bioactive glasses, have been selected for analysis and discussion.
Keywords
- advanced ceramics
- ceramic coatings
- thermal spraying
- coating technologies
- material functionallity
1. Introduction
Beyond polymers and metals, ceramics are one of the three major classes of materials. The word ceramic is originally from the Greek term “keramicos”, that is a burnt material [1]. Nowadays, ceramics are highly employed materials, and according to their properties and applications, such materials are divided into two different categories, i.e., traditional and advanced ceramic materials [1, 2].
On the one hand, there are the traditional ceramics. These materials are entirely based on natural raw materials (clays, micas, quartz and feldspars) and are characterised by non-accurate defined properties and low reproducibility and reliability [2, 3, 4, 5]. This category includes high-volume products such as bricks, tiles and pottery [2, 3, 4, 5].
On the other hand, there are the advanced ceramics, that is, novel ceramic materials synthesised from chemicals of high purity, which exhibit superior properties (particle size distribution, composition, grain size, purity, etc.) tailored to one or various specific applications that require higher performance [2, 3, 4, 5, 6]. This category includes oxides (aluminates, titanites, zirconates, etc.), nitrides, borides and carbides [4].
Both categories also differ in the way in which they are shaped and processed, which usually involves more sophisticated steps for advanced ceramics, as well as in the way they are finished and characterised [1, 2, 4].
Nowadays, traditional ceramic materials still account for a large volume of production in the ceramics industry, but advanced ceramics’ interest has exponentially grown during the last few years [5].
Advanced ceramic materials, also referred to as high-performance ceramics, engineering ceramics or technical ceramics [1, 6], are usually categorised into simple or complex classifications, based on different aspects such as their application, composition or structure [6, 7, 8]. Nevertheless, it could be interesting to divide them from an industrial point of view. According to that, advance ceramics could be grouped into five different categories, as shown in Figure 1 [9].
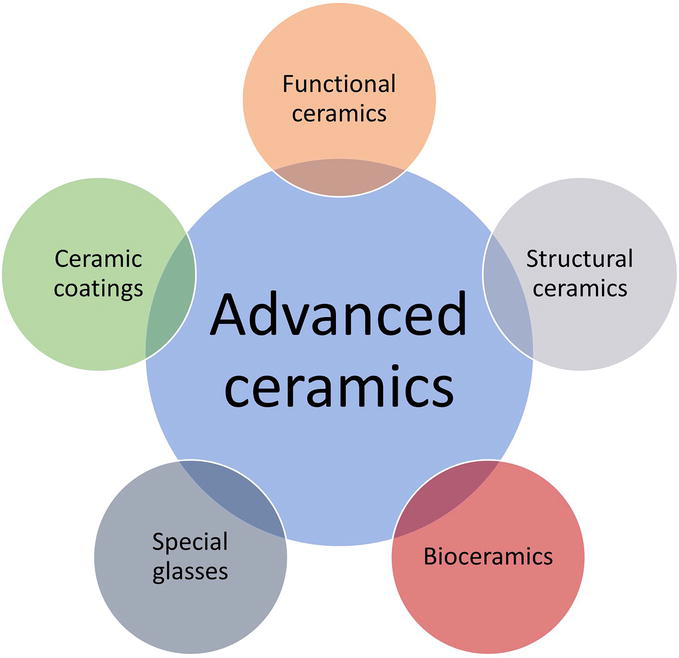
Figure 1.
Possible classification of advanced ceramic materials from an industrial point of view.
Moving in a clockwise direction there are the two largest categories, that is, functional and structural ceramics. The first group includes ceramics for electrical and magnetic applications, while the second involves monoliths and composites of oxides, nitrides, borides and carbides [9]. Then, there are three specific groups, bioceramics (contains materials such as hydroxyapatite, bioactive glasses or alumina), special glasses (intended for optoelectronics applications or withstand fire among others) and ceramic coatings (advanced coatings made from most of the previous materials) [9].
Concerning ceramic coatings, these are typically manufactured through vapour deposition (both physical and chemical), sol–gel deposition and laser processes, but these methods result in low deposition speed coupled with high process costs [9, 10, 11, 12]. Therefore, thermal spraying could be an alternative to these methods since it not only allows the manufacture of ceramic coatings with higher deposition speed and low process costs, but also makes it possible to obtain thicker coatings [10].
The aim of this chapter is to carry out an overview of the field of ceramic coatings’ deposition by thermal spraying based on different examples of advanced ceramics.
2. Fundamentals of thermal spraying
The term thermal spraying encompasses a family of coating manufacturing techniques, in which metals, ceramics, polymers and mixtures of these materials are deposited onto a specific substrate in a fully or partially molten state [13, 14].
In thermal spraying, a given material (also called feedstock) is subjected to an energy source, as shown in Figure 2 [15]. This source takes over the feedstock fragmentation into fine particles and transmission of heat and kinetic energy to them, so that the material is transformed into small droplets in a molten or semi-molten state and accelerated towards the substrate [15]. Then, upon impact on the substrate, these droplets (known as splats) suddenly cool down, flatten and become adhered to the substrate. Finally, the coating builds up by the stacking of the droplets.
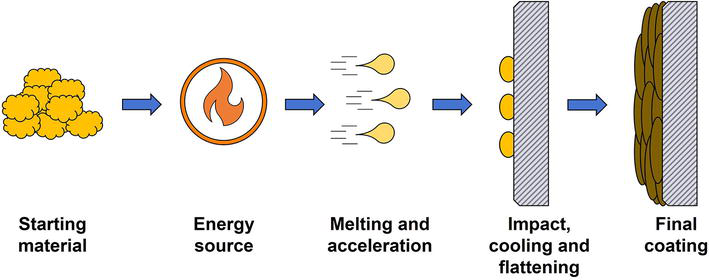
Figure 2.
General process of coatings manufactured by thermal spraying. Figure adapted from [
In addition to the advantages described in the previous section (manufacture of thick coatings, higher deposition speed and low process costs), thermal spraying is a very versatile technology as it can be employed to deposit a large number of materials onto a wide variety of substrates. This is because this technology is capable of fluxing materials with high melting temperature without subjecting the substrate to excessive heating, since the range of temperatures to which the substrate is exposed is very wide (from 100 to 700°C approximately) [14, 16].
Due to its versatility, thermal spraying technology can be used to develop resistant coatings against corrosion, wear and high temperatures for several industrial fields such as power generation, petrochemical, automotive and aerospace among others. Moreover, improvements in both feedstocks and thermal spray processes during the last decades have allowed a significant growth of this technology in new markets, such as biomedical, dielectric and electronic coatings [14, 16].
In thermal spraying, the energy source used can be a flame from a combustion, an electric arc, a thermal plasma or kinetic energy from a decompressed gas [13, 14, 15, 16, 17]. According to the energy source employed, all thermal spraying techniques are classified into four different families [14, 15, 16, 17], as displayed in Figure 3.
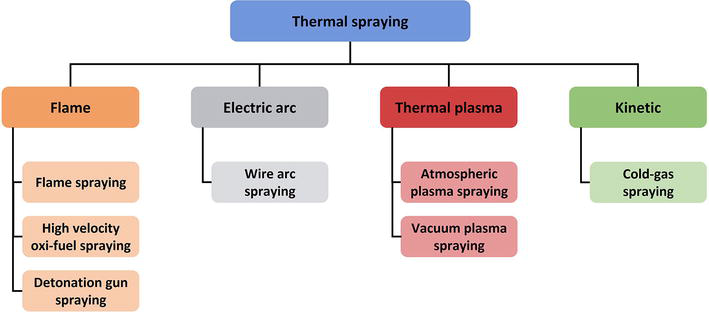
Figure 3.
Thermal spraying family tree according to the energy source. Figure adapted from [
Among all the techniques from the thermal spraying family, advanced ceramics are mainly deposited by plasma spraying [18]. Nevertheless, high velocity oxy-fuel spraying is also starting to receive interest due to the higher density and compactness of the coatings obtained by the high speed at which the material reaches the substrate.
In atmospheric plasma spraying (APS), the energy source is a thermal plasma, which is generated after ionisation of a gaseous mixture (typically argon, hydrogen, helium and mixtures of these) by passing through an electric arc, and recombination of the ions as they exit through a nozzle. The resulting plasma could achieve about 10,000–15,000 K in the hot spot of its core, and once generated, the feedstock is fed directly into the plasma plume where it melts and is accelerated towards the substrate [16, 17].
In high-velocity oxy-fuel spraying (HVOF), a mixture of fuel (typically different hydrocarbon gases and liquids) and oxygen is fed into a continuously pressurised combustion chamber, where it burns at high temperatures. As a result, combustion gases are generated which, due to the pressure difference, are expelled from the combustion chamber and forced through a nozzle where they reach a high velocity. Then, the feedstock is introduced into the stream of combustion gases coming from the combustion chamber, where it melts due to the high temperature of the combustion gases and is accelerated towards the substrate due to the high velocity of the combustion gases [16, 17].
Regardless of the technique, the most employed type of feedstock is powder materials, although over the last two decades the trend in thermal spraying has been towards the use of liquid materials with the aim of obtaining nanostructured coatings with improved properties. The liquid materials used are particle suspensions and solution precursors, and depending on whether one or the other is used, variants of the above techniques emerge, i.e., suspension plasma spraying (SPS), solution precursor plasma spraying (SPPS) and high velocity suspension flame spraying (HVSFS). Suspensions are usually prepared from submicron and nanoparticles using water, ethanol or other organics as solvents, whereas solution precursors are usually made by dissolving alkoxides, salts and acetates in water or ethanol, resulting in purer feedstocks as there are no contaminations from feedstock processing steps such as milling, mixing or spray-drying.
In either method, the behaviour of the material used after being subjected to the corresponding energy source is different. In the case of powders, the material only melts and is accelerated towards the substrate. When injecting the suspension, the material is first split into small suspension droplets. This splitting phenomenon is known as aerodynamic fragmentation and is due to a shear produced on the suspension by the inertia of the plasma plume. The droplet size is influenced by the viscosity of the suspension and the surface tension of the dispersant medium. Immediately after fragmentation, the dispersant starts to evaporate rapidly. From this point on, most of the fine particles contained in the suspension droplets start to sinter instantaneously forming granules, which melt and impact against the substrate to form the coating [19]. Concerning solution precursors, the processes taking place in the plasma plume are even more complex than in the case of suspensions as the material must be formed in the plasma plume itself. When the solution is injected, as in the previous case, the first stages are fragmentation and evaporation of the dispersant medium in microseconds. This is followed by precipitation of the solid in the droplet as the dispersant evaporates. For very small particles, this precipitation is complete over the entire volume of the particle, whereas, for large particles, a crust of material first precipitates on the surface of the droplet and then moves towards the centre of the droplet. Sometimes, the pressure of the liquid inside the droplet causes the initial crust to break and the process starts all over again in the resulting droplet. This is followed by pyrolysis of the precipitated solid and sintering of the formed particles into small granules, which melt and impact against the substrate [19]. The detailed processes for liquid materials correspond to the common cases, although these processes may vary depending on the particle trajectory and the processing parameters used [19].
Both techniques are complex, and the quality and final properties of the manufactured coatings depend on both the temperature and the velocity reached by the material inside the plasma plume, as well as the state of the substrate when the material impacts. At the same time, both temperature and velocity are function of more than 50 parameters. Nonetheless, some of these parameters have a more noticeable effect than the others. Basically, the main process parameters are the type of feedstock, its properties (particle size and flowability for powders and particle size, viscosity and surface tension for liquids) and how it is injected (radially or axially to the plasma plume), the flow rate and type of gases, the input energy (for plasma) and pressure of the chamber (for HVOF), the spraying distance and the state of the substrate (surface temperature and topography) [16, 17].
3. Manufacture of thermally sprayed ceramic coatings
3.1 Alumina coatings
Alumina or aluminium oxide (Al2O3) is a cheap and hard advanced ceramic, which has good wear, high hardness and corrosion, electric and thermal resistance [20]. Consequently, it is one of the most flexible and attractive advanced ceramics used in thermal spraying to be deposited onto metallic substrates for wear and erosion resistance, corrosion protection and both thermal and electrical insulation [21, 22, 23].
The manufacture of this kind of coating is commonly done by APS from powder feedstocks, although HVOF is also studied [24, 25]. In fact, with the utilisation of HVOF, the resulting coatings are less porous and compacted, and hence harder and tougher with higher electrical resistivity [20, 22, 24, 26], whereas with APS a more porous coating is obtained with better thermal insulation [26].
Regardless of the spraying technique, the main drawback of employing this oxide is that the resulting coatings are mainly composed of metastable phases, such as γ-Al2O3 and δ-Al2O3, which negatively affect the long-term stability of the as-sprayed coatings and their final properties, especially in humid environments (corrosion protection in marine) and electrical insulation applications, especially APS coatings [22, 26].
Several attempts were made to increase the α-phase present in the as-sprayed coatings, including post-heat treatments at temperatures around 1200–1300°C (unsuitable temperatures for many metallic substrates) or alloying with other oxides [27, 28]. For that purpose, chromia is the most employed oxide since an addition of 20 wt% of chromic oxide (Cr2O3) gives rise to a solid solution with Al2O3 in the α-modification [29].
In the last decade, it has been found that with the utilisation of liquid feedstocks, instead of powders, it is possible to retain a higher amount of α-phase (up to 70%) without the necessity of a posttreatment [30, 31]. Moreover, the electrical, mechanical properties and wear behaviour could be improved using this type of feedstock [32, 33], when compared with the coatings manufactured by conventional thermal spraying methods based on powder feedstocks.
Finally, alumina is also sprayed with zirconia to increase the toughness of the coatings and with titania to improve mechanical and tribological properties [34, 35, 36, 37].
3.2 Yttria-stabilised zirconia coatings
Zirconia or zirconium oxide (ZrO2) could be a suitable candidate to be used in the aerospace, automotive and power generation sectors to manufacture what is known as thermal barrier coatings (TBCs), that is, thermally sprayed ceramic coatings used to protect different metallic components of turbines and engines against corrosion and oxidation and enhance their durability and efficiency [38, 39].
This advanced ceramic has gained interest due to its excellent shock resistance, low thermal conductivity and moderate coefficaient of thermal expansion (compared to other ceramics) [39, 40].
Despite all of this, the use of pure zirconia in high temperature applications is limited due to its polymorphism, as shown in Figure 4. During cooling, the transformation from tetragonal phase to monoclinic phase leads to a volume reduction of approximately 4%, which results in the fracture and delamination of the coating [40, 41].

Figure 4.
Phase transformations of ZrO2 during cooling [
Consequently, a lattice stabilising agent is used, blocking the transformation from tetragonal to monoclinic and giving rise to a metastable tetragonal phase without volume change [41]. These agents are usually divalent, trivalent or tetravalent cations such as Ca+2, Y+3 or Ce+4, which are introduced in different quantities depending on the phase to be obtained, whose purpose is to generate oxygen vacancies in the crystalline network until the coordination number of Zr is reduced from 8 to around 7.5 [42]. In the case of thermal spraying, the most common agent used is yttrium oxide (Y2O3), due to the low amount needed to stabilise the compound, and its amount varies between 7 and 9 wt% (≈4 mol%) as the desired phase is the tetragonal one (which presents the lowest thermal conductivity, i.e., ≈2 W/m K) [42, 43, 44, 45]. The resulting material is called yttria-stabilised zirconia or YSZ.
Among the thermal spraying techniques, TBCs are usually manufactured by APS from powder feedstocks [46, 47]. Employing this method, a coating is obtained with a lamellar or layered structure due to the stacking of the splats. In addition, the latter are separated by intersplat pores resulting from their rapid solidification, very fine voids formed by incomplete splat contact or presence of unmelted particles and cracks due to thermal stresses [46, 47]. Although this type of microstructure could be suitable for reducing the thermal conductivity of the coating, it negatively affects its performance, especially the thermal cycle life of the coating which is limited by insufficient strain compliance [47]. Moreover, improvement of the efficiency of gas turbines by further increases of the combustion and cooling technology in combination with higher turbine inlet temperatures implies that yttria-stabilised zirconia (4 mol% yttria) is confronted with certain limitations due to sintering and phase transformation [48]. As a result, both thermal conductivity and stiffness of the coating increase, resulting in acceleration of the spallation of the coating [48, 49].
With the aim of enhancing the TBCs’ performance, the focus has been on the modification or improvement of the coating microstructure. One first approach was the deposition of coatings from submicron and nanostructured powders introduced in the plasma plume as spray-dried granules obtained from suspensions [50, 51, 52]. Coatings with a bimodal microstructure were obtained, that is, partially melted agglomerates (maintaining nanoparticles from the starting feedstock) surrounded by fully melted areas which act as matrix with superior properties compared with the conventional APS coatings from micrometric powders [52].
However, the definitive approach was the introduction of liquid feedstocks into the field of thermal spraying, and instead of spray-drying particle suspensions, these and precursor solutions began to be directly deposited by SPS and SPPS. Despite the low deposition rate, using these techniques, lower thermal conductivities were obtained compared to APS coatings [19]. In addition, by employing liquid feedstocks a totally new microstructure was obtained which is known as the “columnar structure”. According to Vanevery et al. [53], when particles less than ≈3 μm in size are used, after melting and before reaching the substrate, the plasma drag forces during substrate impingement dominate the droplet inertia and redirect the droplet velocity from normal to along to the substrate surface. Consequently, droplets impact preferentially on asperities, generating deposits that grow to become columnar structures separated by linear porosity bands, which improve the performance of the coating as they stop the propagation of transversal cracks. Moreover, this effect is more marked when ethanol or organics are used as solvents, as they possess lower surface tension than water and hence, the liquid drop injected into the plasma torch will be less in size, resulting in a few number of agglomerated particles after evaporation of the solvent.
Other ways to improve the performance of the TBCs are by doping the YSZ with rare earths or by mixing YSZ and zirconates with pyrochlore structure, producing TBCs with even lower thermal conductivity and higher stability at temperature [19]. In both cases, the utilisation of SPS and SPPS facilitates this task, as both produce thinner layers than APS. Moreover, the employment of solution precursors highly facilitates the modification of the composition.
Regarding the doping of YSZ, typical dopants used are Nd2O3, Yb2O3 or Gd2O3 [19]. Nitrate precursor of the desired rare earth is commonly dissolved into the YSZ suspension or mixed with the precursors employed for YSZ. This gives a YSZ matrix with the rare earth oxides embedded inside as defects. Besides, YSZ-doped coatings can also be manufactured by hybrid plasma spraying, where a powder feedstock (YSZ) and a liquid feedstock (rare earth precursor) are deposited at the same time but are injected along different paths and at different points of the plasma plume [49].
Concerning the zirconates, the most employed ones are gadolinium zirconate (Gd2Zr2O7) and lanthanum zirconate (La2Zr2O7) [19]. Both materials present lower thermal conductivity, higher melting point, higher sintering resistance and higher phase stability than YSZ. Their drawback is their very low thermal expansion coefficient, preventing the use of these materials on their own [19]. Therefore, zirconates are deposited in combination with YSZ as intermediate layer using two types of layouts as shown in Figure 5, i.e., multi-layered coatings, in which layers of each material are deposited, and functionally graded coatings, where the composition is gradually modified from the bottom of the coating to the top of the coating.
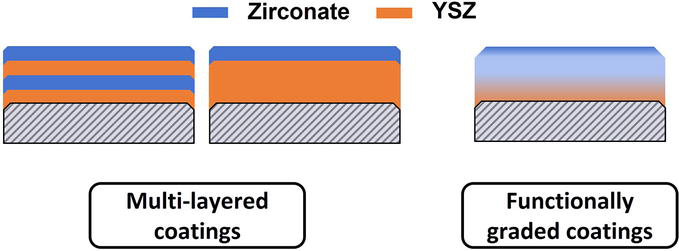
Figure 5.
Layout of multi-layered and functionally graded coatings.
However, functionally graded coatings present better performance compared to multi-layered, since the latter may result in failures due to thermal expansion mismatch between the layers [49].
Another application of yttria-stabilised zirconia (8 mol% yttria) is to employ it as a coating for metal-supported solid oxide fuel cells (SOFCs), more concretely covering the electrolytes [54]. Several attempts were made to deposit this type of coating by APS and high velocity oxy-fuel (HVOF). However, the utilisation of SPS and SPPS results in thinner and more uniform electrolyte coatings [55].
3.3 Zirconium silicate coatings
Zirconium silicate or zircon (ZrSiO4) is a ceramic material which can be found naturally in zircon sands. Zircon could be a cheap and suitable candidate to replace yttria-stabilised zirconia in thermal barrier coatings as it possesses excellent thermal shock resistance, low thermal expansion coefficient and low thermal conductivity [56, 57]. Furthermore, its dielectric properties, corrosion resistance and the fact of being chemically inert at low and high temperatures allow its use in electrical applications and as an environmental barrier coating (EBC), respectively [58, 59].
Zircon coatings are typically manufactured from powder feedstocks by plasma spraying [57, 60, 61, 62, 63]. The main problem of using this material is that zircon decomposes in ZrO2 and SiO2 due to the high temperatures of the plasma plume, and the fast cooling prevents the recombination of both oxides. In addition, the different melting temperatures of ZrO2 and SiO2 give rise to a coating composed of crystalline ZrO2 (tetragonal and monoclinic as it is not stabilised) as a major phase, a glassy solidified SiO2 as a secondary phase and no zircon or only a small amount as a residual phase [56, 57, 58, 59, 60, 61, 62, 63].
Recently, a novel approach was done based on liquid feedstocks. Aqueous suspensions of zircon particles were prepared and deposited by SPS modifying different parameters related to the technique [64]. It has been found that, due to the removal of part of the energy of the plasma plume to evaporate the water, a significant proportion of the starting zircon is preserved in the final microstructure, which remains around 20%, regardless of the processing conditions selected [64].
3.4 Hydroxyapatite and bioactive glass coatings
Coatings from bioceramic materials can also be manufactured by thermal spraying. Concretely, in load-bearing applications such as dental and orthopaedic metallic implants where the biomaterials cannot be used by themselves due to their brittleness. Thus, a composite is obtained that combines good mechanical properties (provided by the metallic substrate) with good osseointegration (provided by the osseoinductive ceramic layer), hence avoiding corrosion and encapsulation of the implant by fibrous tissue.
For that purpose, the most employed bioceramic is synthetic hydroxyapatite or HAp, with a stoichiometric chemical formula of Ca10(PO4)6(OH)2, a molar Ca:P ratio of 1.67 and a composition close to the mineral phase of bone [65, 66]. Coatings of HA are typically deposited by APS and HVOF from powder feedstocks [65, 66, 67, 68]. In fact, these techniques are approved by the US Food and Drug Administration (FDA) for the deposition of HA coatings [65, 67].
Since these coatings do not require high thickness to develop its function, they are easily deposited by APS. The most important drawback of plasma-sprayed HAp coatings is to maintain the purity and crystallinity of the initial feedstock in the as-sprayed coatings. Due to the high temperatures in plasma spraying, HAp decomposes and suffers partial dehydroxylation during the manufacture of the coatings, and due to the rapid cooling it results in a deposited layer that combines crystalline HAp, amorphous phase and different calcium phosphates (tricalcium phosphate, tetracalcium phosphate and calcium oxide) [69, 70].
Both amorphous phase and calcium phosphates are undesirable, as they increase the dissolution rate and resorption of the coating, leading to a failure of the coating before it can perform its intended function and negatively affect coating’s fixation to the implant and their long-term stability [69, 70]. Although several attempts were done by different researchers by modifying the most important parameters of APS, it was not possible to obtain a fully crystalline HAp coating [71, 72]. Only after a posttreatment, the full crystallinity was reached [72].
High velocity oxy-fuel (HVOF) has also been employed to deposit HAp coatings as an alternative to APS [73, 74, 75, 76]. The lower temperatures and higher velocities of this method give rise to denser, less porous and better adhered coatings than in APS with higher crystallinity. However, it is still needed to conduct a posttreatment in order to reach fully crystalline HAp coating [76].
In both methods (APS and HVOF), it is also common to deposit HAp with other materials such as Ti, TiO2 or ZrO2 to improve wear resistance adhesion and fracture toughness [73, 77, 78, 79, 80].
As in the other materials exposed in previous sections, HAp was also affected by the introduction of liquid feedstocks in the thermal spraying field, being this material deposited by means of SPS, SPPS and HVSFS [68, 81, 82, 83, 84, 85, 86, 87, 88].
When depositing coatings by SPS, it is possible to obtain ceramic layers with a higher degree of crystallinity than the APS counterparts. This is mainly due to the trajectory of the particles in the plasma plume and the lower energy reaching them due to the evaporation of water (in the case of using this solvent) [81, 82, 83]. Regarding the trajectory, as the particles are lower in size they are more easily affected by the drag forces of the plasma plume, so that not all of them penetrate the centre of the plume, especially when the material is injected radially. As a result, a coating composed of two different zones is obtained, a dense zone formed from melted particles and a sintered zone composed of grain which were sintered instead of melting during flying across the plasma plume, preserving the crystallinity of the HAp feedstock [81, 82]. Furthermore, the utilisation of HVFSF gives rise to higher crystallinity compared with APS coatings, although the deposition efficiency is much lower [68].
Moreover, when SPPS was employed, deposition efficiency was much lower than in SPS, but the resulting coatings present higher crystallinity than those deposited by APS [86, 87]. Nevertheless, Aruna et al. have proven that SPS HAp coatings continue to be more promising than SPPS [88].
Finally, the utilisation of liquid feedstocks, especially solution precursors, greatly facilitates the modification of the composition with the aim of adding dopant ions such as Cu+2 or Zn+2 to improve the performance of the as-sprayed coatings [89, 90].
Bioactive glasses are the other type of bioaceramic that is under study for the deposition of bioactive coatings by means of thermal spraying. The term bioactive glass refers to a vitreous material that is biocompatible with tissues and body fluids as well as being non-carcinogenic, non-mutagenic and non-antigenic [91]. The first glass in this category is known as bioactive glass 45S5 whose composition is 45.0% SiO2, 24.5% CaO, 24.5% Na2O and 6.0% P2O5 (in wt%) [91].
From this glass, several researchers have developed variants over time by slightly modifying the composition of this glass (substitution and/or elimination of oxides) depending on the application or method of synthesis. Some examples are the bioactive glass 58S [92], without sodium, as it is typically synthesised by the sol–gel method, the Bio-K [93], which includes potassium instead of sodium in order to reduce the crystallisation tendency of the glass, or the BGMS10 [94], which includes magnesium and strontium to highly increase the crystallisation temperature.
These glasses are emerging as substitutes for HAp in the manufacture of coatings by thermal spraying, since, although both materials have good biocompatibility and similar mechanical properties [95], bioactive glasses have a higher bioactivity index [95, 96], are osteoinductive, osteoconductive and osseointegrative, and during deposition by thermal spraying, they maintain their amorphous character (a requirement for their bioactivity) [65].
Like HAp, these glasses are deposited by different techniques, i.e., APS, SPS, HVSFS and SPPS [94, 97, 98, 99, 100, 101, 102]. The use of liquid materials results in more porous and rougher microstructures, which increase the bioactivity of the coatings. However, there is still a lot of research ahead since, as indicated in two very recent reviews [103, 104], regardless of the spraying technique employed, the long-term stability of this kind of coating is not satisfactory yet and their adhesion to the substrate must be improved.
4. Conclusions and future trends in thermally sprayed ceramics
In this chapter, a brief overview of the progress and current state of different thermal sprayed ceramics has been carried out. From the previous sections, it can be seen how thermal spraying can play an important role in obtaining coatings from advanced ceramic materials. After discussing several examples of materials, it can be appreciated how it is possible to obtain different microstructures and properties depending on the spraying technique used. However, these techniques are so complex and involve so many variables. Therefore, it is very difficult to decide which technique is the definitive one for each material, as this depends on the application for which the coating is intended.
By contrast, the use of liquid feedstocks (particle suspensions and solution precursors) results in all cases in an improvement of both the microstructure and properties of the coatings. However, there is still a lot of research work to be done in order to fully understand the interaction between liquid feedstocks and the energy source used for their deposition, especially in the case of solution precursors, the effect of this interaction on the microstructure obtained and how it relates to the final properties, the aim of being able to scale the employment of these materials at industrial level.
Therefore, it could be said that the future of thermal spraying lies in the use of liquid filler materials, especially precursor solutions, since their synthesis is simpler from a processing point of view and results in materials whose composition is easier to modify and much purer.
Acknowledgments
Authors of the present work would like to acknowledge for the grants PID2021-128548OB-C21 funded by MCIN/AEI/10.13039/501100011033 and “ERDF A way of making Europe”, the Margarita Salas postdoctoral contract of Eugeni Cañas (MGS/2022/20) financed by the European Union—NextGenerationEU and Grant CIGE/2021/076 funded by Generalitat Valenciana.
References
- 1.
Ayode Otitoju T, Ugochukwu Okoye P, Chen G, et al. Advanced ceramic components: Materials, fabrication, and applications. Journal of Industrial and Engineering Chemistry. 2020; 85 :34-65 - 2.
Carter CB, Norton MG. Ceramic Materials: Science and Engineering. New York: Springer; 2013 - 3.
Heimann RB. Classic and Advanced Ceramics: From Fundamentals to Applications. Weinheim: Wiley-VCH; 2010 - 4.
Salamon D. Advanced Ceramics. 1st ed. Waltham: Butterworth-Heinemann; 2014 - 5.
Rahaman MN. Ceramic Processing and Sintering. 2nd ed. Boca Ratón: CRC Press; 2010 - 6.
Lakhdar Y, Tuck C, Binner J, et al. Additive manufacturing of advanced ceramic materials. Progress in Materials Science. 2021; 116 :100736 - 7.
Kurian M, Thankachan S. Introduction: Ceramics classification and applications. In: Kurian M, Thankachan S, Nair SS, editors. Ceramic Catalysts: Materials, Synthesis and Applications. Amsterdam: Elsevier; 2023. pp. 1-17 - 8.
Riedel R, Chen IW, editors. Ceramics Science and Technology. Vol. 1. Structures. Weinheim: Wiley-VCH; 2008 - 9.
Rödel J, Kounga ABN, Weissenberger-Eibl M, et al. Development of a roadmap for advanced ceramics: 2010-2025. Journal of the European Ceramic Society. 2009; 29 :1549-1560 - 10.
Kassner H, Siegert R, Hathiramani D, et al. Application of suspension plasma spraying (SPS) for manufacture of ceramic coatings. Journal of Thermal Spray Technology. 2008; 17 :115-123 - 11.
Zanurin A, Johari NA, Alias J, et al. Research progress of sol-gel ceramic coating: A review. Materials Today Proceedings. 2022; 48 :1849-1854 - 12.
Horcher A, Tangermann-Gerk K, Krenkel W, et al. Advanced ceramic coatings on aluminum by laser treatment of filled organosilazane-based composites. Ceramics International. 2022; 48 :23284-23292 - 13.
Fauchais P, Vardelle A, Dussoubs B. Quo Vadis thermal spraying? Journal of Thermal Spray Technology. 2001; 10 :44-66 - 14.
Amin S, Panchal H. A Review on thermal spray coating processes. International Journal of Current Trends in Engineering & Research. 2016; 2 :556-563 - 15.
Davis J, editor. Handbook of Thermal Spray Technology. Ohio: ASM International; 2004 - 16.
Espallargas N, editor. Future Development of Thermal Spray Coatings: Types, Designs, Manufacture and Applications. Cambirdge: Woodhead Publishing; 2015 - 17.
Pawlowski L. The Science and Engineering of Thermal Spray Coatings. 2nd ed. Chichester: John Wiley and Sons; 2008 - 18.
Li CJ, Luo XT, Yao SW, et al. The bonding formation during thermal spraying of ceramic coatings: A review. Journal of Thermal Spray Technology. 2022; 31 (4):780-817 - 19.
Fan W, Bai Y. Review of suspension and solution precursor plasma sprayed thermal barrier coatings. Ceramics International. 2016; 42 :14299-14312 - 20.
Matikainen V, Niemi K, Koivuluoto H, et al. Abrasion, erosion and cavitation erosion wear properties of thermally sprayed alumina based coatings. Coatings. 2014; 4 :18-36 - 21.
Lampke T, Meyer D, Alisch G, et al. Alumina coatings obtained by thermal spraying and plasma anodising—A comparison. Surface & Coatings Technology. 2011; 206 :2012-2016 - 22.
Toma FL, Scheitz S, Berger LM, et al. Comparative study of the electrical properties and characteristics of thermally sprayed alumina and spinel coatings. Journal of Thermal Spray Technology. 2011; 20 :195-204 - 23.
Psyllaki PP, Jeandin M, Pantelis DI. Microstructure and wear mechanisms of thermal-sprayed alumina coatings. Materials Letters. 2001; 47 :77-82 - 24.
Bolelli G, Lusvarghi L, Manfredini T, et al. Comparison between plasma- and HVOF-sprayed ceramic coatings. Part I: Microstructure and mechanical properties. International Journal of Surface Science and Engineering. 2007; 1 :38-61 - 25.
Michalak M, Sokołowski P, Szala M, et al. Wear behavior analysis of Al2O3 coatings manufactured by APS and HVOF spraying processes using powder and suspension feedstocks. Coatings. 2021; 11 :879 - 26.
Shakhova I, Mironov E, Azarmi F, et al. Thermo-electrical properties of the alumina coatings deposited by different thermal spraying technologies. Ceramics International. 2017; 43 :15392-15401 - 27.
Krishnan R, Dash S, Sole RK, et al. Fabrication and characterisation of laser surface modified plasma sprayed alumina coatings. Surface Engineering. 2013; 18 :208-212 - 28.
Stahr CC, Saaro S, Berger LM, et al. Dependence of the stabilization of α-alumina on the spray process. Journal of Thermal Spray Technology. 2007; 16 :822-830 - 29.
Chráska P, Dubsky J, Neufuss K, et al. Alumina-base plasma-sprayed materials part I: Phase stability of alumina and alumina-chromia. Journal of Thermal Spray Technology. 1997; 6 :320-326 - 30.
Toma FL, Berger LM, Stahr CC, et al. Microstructures and functional properties of suspension-sprayed Al2O3 and TiO2 coatings: An overview. Journal of Thermal Spray Technology. 2010; 19 :262-274 - 31.
Sivakumar G, Dusane RO, Joshi SV. A novel approach to process phase pure α-Al2O3 coatings by solution precursor plasma spraying. Journal of the European Ceramic Society. 2013; 33 :2823-2829 - 32.
Toma FL, Berger LM, Scheitz S, et al. Comparison of the microstructural characteristics and electrical properties of thermally sprayed Al2O3 coatings from aqueous suspensions and feedstock powders. Journal of Thermal Spray Technology. 2012; 21 :480-488 - 33.
Michalak M, Latka L, Sokolowski P, et al. Microstructural, mechanical and tribological properties of finely grained Al2O3 coatings obtained by SPS and S-HVOF methods. Surface & Coatings Technology. 2020; 404 :126463 - 34.
Kiilakoski J, Musalek R, Lukac F, et al. Evaluating the toughness of APS and HVOF-sprayed Al2O3-ZrO2-coatings by in-situ- and macroscopic bending. Journal of the European Ceramic Society. 2018; 38 :1908-1918 - 35.
Carpio P, Salvador MD, Borrell A, et al. Alumina-zirconia coatings obtained by suspension plasma spraying from highly concentrated aqueous suspensions. Surface & Coatings Technology. 2016; 307 :713-719 - 36.
Thalib Basha GM, Srikanth A, Venkateshwarlu B. A critical review on nano structured coatings for alumina-titania (Al2O3-TiO2) deposited by air plasma spraying process (APS). Materials Today Proceedings. 2020; 22 :1554-1562 - 37.
Jordan EH, Gell M, Sohn YH, et al. Fabrication and evaluation of plasma sprayed nanostructured alumina–titania coatings with superior properties. Materials Science and Engineering: A. 2001; 301 :80-89 - 38.
Ghasemi R, Vakilifard H. Plasma-sprayed nanostructured YSZ thermal barrier coatings: Thermal insulation capability and adhesion strength. Ceramics International. 2017; 43 :8556-8563 - 39.
Schlichting KW, Padture NP, Klemens PG. Thermal conductivity of dense and porous yttria-stabilized zirconia. Journal of Materials Science. 2001; 36 :3003-3010 - 40.
Tian YS, Chen CZ, Wang DY, et al. Recent developments in zirconia thermal barrier coatings. Surface Review and Letters. 2005; 12 :369-378 - 41.
Hannink RHJ, Kelly PM, Muddle BC. Transformation toughening in zirconia-containing ceramics. Journal of the American Ceramic Society. 2000; 83 :461-487 - 42.
Tsipas SA. Effect of dopants on the phase stability of zirconia-based plasma sprayed thermal barrier coatings. Journal of the European Ceramic Society. 2010; 30 :61-72 - 43.
Moskal G. Criteria of assessment of powders provided to spray by the APS method for new and conventional layers type TBC. Archives of Materials Science and Engineering. 2009; 27 :29-36 - 44.
Clarke DR, Phillpot SR. Thermal barrier coating materials. Materials Today. 2005; 8 :22-29 - 45.
Hayashi H, Saitou T, Maruyama N, et al. Thermal expansion coefficient of yttria stabilized zirconia for various yttria contents. Solid State Ionics. 2005; 176 :613-619 - 46.
Chen LB. Yttria-stabilized zirconia thermal barrier coatings—A review. Surface Review and Letters. 2006; 13 :535-544 - 47.
Huang JB, Wang WZ, Li YJ, et al. A novel strategy to control the microstructure of plasma-sprayed YSZ thermal barrier coatings. Surface & Coatings Technology. 2020; 402 :126304 - 48.
Vaßen R, Jarligo MO, Steinke T, et al. Overview on advanced thermal barrier coatings. Surface & Coatings Technology. 2010; 205 :938-942 - 49.
Joshi SV, Sivakumar G, Raghuveer T, et al. Hybrid plasma-sprayed thermal barrier coatings using powder and solution precursor feedstock. Journal of Thermal Spray Technology. 2014; 23 :616-624 - 50.
Carpio P, Borrell A, Salvador MD, et al. Microstructure and mechanical properties of plasma spraying coatings from YSZ feedstocks comprising nano- and submicron-sized particles. Ceramics International. 2015; 41 :4108-4117 - 51.
Loghman-Estarki MR, Pourbafrany M, Shoja Razavi R, et al. Preparation of nanostructured YSZ granules by the spray drying method. Ceramics International. 2014; 40 :3721-3729 - 52.
Carpio P, Bannier E, Salvador MD, et al. Multilayer and particle size-graded YSZ coatings obtained by plasma spraying of micro- and nanostructured feedstocks. Journal of Thermal Spray Technology. 2014; 23 :1362-1372 - 53.
Vanevery K, Krane MJM, Trice RW, et al. Column formation in suspension plasma-sprayed coatings and resultant thermal properties. Journal of Thermal Spray Technology. 2011; 20 :817-828 - 54.
Marr M, Kuhn J, Metcalfe C, et al. Electrochemical performance of solid oxide fuel cells having electrolytes made by suspension and solution precursor plasma spraying. Journal of Power Sources. 2014; 245 :398-405 - 55.
Marr M, Kesler O. Permeability and microstructure of suspension plasma-sprayed YSZ electrolytes for SOFCs on various substrates. Journal of Thermal Spray Technology. 2012; 21 :1334-1346 - 56.
Schelz S, Enguehard F, Caron N, et al. Recombination of silica and zirconia into zircon by means of laser treatment of plasma-sprayed coatings. Journal of Materials Science. 2008; 43 :1948-1957 - 57.
Ramaswamy P, Seetharamu S, Varma KBR, et al. Thermal barrier coating application of zircon sand. Journal of Thermal Spray Technology. 1999; 8 :447-453 - 58.
Ctibor P, Pala Z, Nevrlá B, et al. Plasma-sprayed fine-grained zirconium silicate and its dielectric properties. Journal of Materials Engineering and Performance. 2017; 26 :2388-2393 - 59.
Liu L, Zheng W, Ma Z, et al. Study on water corrosion behavior of ZrSiO4 materials. Journal of Advanced Ceramics. 2018; 7 :336-342 - 60.
Chráska P, Neufuss K, Herman H. Plasma spraying of zircon. Journal of Thermal Spray Technology. 1997; 6 :445-448 - 61.
Rudajevová A. Thermal properties of plasma-sprayed ZrSiO4 material. Surface & Coatings Technology. 1994; 64 :47-51 - 62.
Suzuki M, Sodeoka S, Inoue T. Zircon-based ceramics composite coating for environmental barrier coating. Journal of Thermal Spray Technology. 2008; 17 :404-409 - 63.
Suzuki M, Sodeoka S, Inoue T. Structure control of plasma sprayed zircon coating by substrate preheating and post heat treatment. Materials Transactions. 2005; 46 :669-674 - 64.
Cañas E, Rosado E, Alcázar C, et al. Challenging zircon coatings by suspension plasma spraying. Journal of the European Ceramic Society. 2022; 42 :4369-4376 - 65.
Juhasz JA, Best SM. Bioactive ceramics: Processing, structures and properties. Journal of Materials Science. 2011; 47 (2):610-624 - 66.
Berndt C, Fahad Hasan M, Tietz U, et al. A review of hydroxyapatite coatings manufactured by thermal spray. In: Ben-Nissan B, editor. Advances in Calcium Phosphate Biomaterials. Berlin, Heidelberg: Springer; 2014. pp. 267-329 - 67.
Balani K, Chen Y, Harimkar SP, et al. Tribological behavior of plasma-sprayed carbon nanotube-reinforced hydroxyapatite coating in physiological solution. Acta Biomaterialia. 2007; 3 :944-951 - 68.
Gadow R, Killinger A, Stiegler N. Hydroxyapatite coatings for biomedical applications deposited by different thermal spray techniques. Surface & Coatings Technology. 2010; 205 :1157-1164 - 69.
Sun L, Berndt CC, Gross KA, et al. Material fundamentals and clinical performance of plasma-sprayed hydroxyapatite coatings: A review. Journal of Biomedical Materials Research. 2001; 58 :570-592 - 70.
Cheang P, Khor KA. Addressing processing problems associated with plasma spraying of hydroxyapatite coatings. Biomaterials. 1996; 17 :537-544 - 71.
Sun L, Berndt CC, Grey CP. Phase, structural and microstructural investigations of plasma sprayed hydroxyapatite coatings. Materials Science and Engineering: A. 2003; 360 :70-84 - 72.
Xue W, Tao S, Liu X, et al. In vivo evaluation of plasma sprayed hydroxyapatite coatings having different crystallinity. Biomaterials. 2004; 25 :415-421 - 73.
Henao J, Cruz-bautista M, Hincapie-Bedoya J, et al. HVOF hydroxyapatite/titania-graded coatings: Microstructural, mechanical, and in vitro characterization. Journal of Thermal Spray Technology. 2018; 27 :1302-1321 - 74.
Lima RS, Khor KA, Li H, et al. HVOF spraying of nanostructured hydroxyapatite for biomedical applications. Materials Science and Engineering: A. 2005; 396 :181-187 - 75.
Khor KA, Li H, Cheang P. Significance of melt-fraction in HVOF sprayed hydroxyapatite particles, splats and coatings. Biomaterials. 2004; 25 :1177-1186 - 76.
Fernández J, Gaona M, Guilemany JM. Effect of heat treatments on HVOF hydroxyapatite coatings. Journal of Thermal Spray Technology. 2007; 16 :220-228 - 77.
Zheng X, Huang M, Ding C. Bond strength of plasma-sprayed hydroxyapatite/Ti composite coatings. Biomaterials. 2000; 21 :841-849 - 78.
Ong JL, Carnes DL, Bessho K. Evaluation of titanium plasma-sprayed and plasma-sprayed hydroxyapatite implants in vivo. Biomaterials. 2004; 25 :4601-4606 - 79.
Li H, Khor KA, Cheang P. Titanium dioxide reinforced hydroxyapatite coatings deposited by high velocity oxy-fuel (HVOF) spray. Biomaterials. 2002; 23 :85-91 - 80.
Li H, Khor KA, Kumar R, et al. Characterization of hydroxyapatite/nano-zirconia composite coatings deposited by high velocity oxy-fuel (HVOF) spray process. Surface & Coatings Technology. 2004; 182 :227-236 - 81.
Kozerski S, Pawlowski L, Jaworski R, et al. Two zones microstructure of suspension plasma sprayed hydroxyapatite coatings. Surface & Coatings Technology. 2010; 204 :1380-1387 - 82.
Łatka L, Pawlowski L, Chicot D, et al. Mechanical properties of suspension plasma sprayed hydroxyapatite coatings submitted to simulated body fluid. Surface & Coatings Technology. 2010; 205 :954-960 - 83.
Zheng B, Luo Y, Liao H, et al. Investigation of the crystallinity of suspension plasma sprayed hydroxyapatite coatings. Journal of the European Ceramic Society. 2017; 37 :5017-5021 - 84.
Bolelli G, Bellucci D, Cannillo V, et al. Suspension thermal spraying of hydroxyapatite: Microstructure and in vitro behaviour. Materials Science and Engineering: C. 2014; 34 :287-303 - 85.
Krieg P, Killinger A, Gadow R, et al. High velocity suspension flame spraying (HVSFS) of metal doped bioceramic coatings. Bioactive Materials. 2017; 2 :169 - 86.
Candidato RT, Sokołowski P, Pawłowski L, et al. Development of hydroxyapatite coatings by solution precursor plasma spray process and their microstructural characterization. Surface & Coatings Technology. 2017; 318 :39-49 - 87.
Huang Y, Song L, Huang T, et al. Characterization and formation mechanism of nano-structured hydroxyapatite coatings deposited by the liquid precursor plasma spraying process. Biomedical Materials. 2010; 5 :054113 - 88.
Aruna ST, Kulkarni S, Chakraborty M, et al. A comparative study on the synthesis and properties of suspension and solution precursor plasma sprayed hydroxyapatite coatings. Ceramics International. 2017; 43 :9715-9722 - 89.
Unabia RB, Bonebeau S, Candidato RT, et al. Investigation on the structural and microstructural properties of copper-doped hydroxyapatite coatings deposited using solution precursor plasma spraying. Journal of the European Ceramic Society. 2019; 39 :4255-4263 - 90.
Candidato RT, Sergi R, Jouin J, et al. Advanced microstructural study of solution precursor plasma sprayed Zn doped hydroxyapatite coatings. Journal of the European Ceramic Society. 2018; 38 :2134-2144 - 91.
Gurbinder K. Bioactive Glasses–Potential Biomaterials for Future Therapy. 1st ed. Switzerland: Springer Nature; 2017 - 92.
Jones JR. Review of bioactive glass: From Hench to hybrids. Acta Biomaterialia. 2013; 9 :4457-4486 - 93.
Cannillo V, Sola A. Potassium-based composition for a bioactive glass. Ceramics International. 2009; 35 :3389-3393 - 94.
Cañas E, Orts MJ, Sánchez E, et al. Deposition of bioactive glass coatings based on a novel composition containing strontium and magnesium. Journal of the European Ceramic Society. 2022; 42 :6213-6221 - 95.
Goller G, Demirkiran H, Oktar FN, et al. Processing and characterization of bioglass reinforced hydroxyapatite composites. Ceramics International. 2003; 29 :721-724 - 96.
Lefebvre L, Chevalier J, Gremillard L, et al. Structural transformations of bioactive glass 45S5 with thermal treatments. Acta Materialia. 2007; 55 :3305-3313 - 97.
Cannillo V, Sola A. Different approaches to produce coatings with bioactive glasses: Enamelling vs plasma spraying. Journal of the European Ceramic Society. 2010; 30 :2031-2039 - 98.
Calvo VL, Cabedo MV, Bannier E, et al. 45S5 bioactive glass coatings by atmospheric plasma spraying obtained from feedstocks prepared by different routes. Journal of Materials Science. 2014; 49 :7933-7942 - 99.
Cattini A, Łatka L, Bellucci D, et al. Suspension plasma sprayed bioactive glass coatings: Effects of processing on microstructure, mechanical properties and in-vitro behaviour. Surface & Coatings Technology. 2013; 220 :52-59 - 100.
Cañas E, Vicent M, Orts MJ, et al. Bioactive glass coatings by suspension plasma spraying from glycolether-based solvent feedstock. Surface & Coatings Technology. 2017; 318 :190-197 - 101.
Altomare L, Bellucci D, Bolelli G, et al. Microstructure and in vitro behaviour of 45S5 bioglass coatings deposited by high velocity suspension flame spraying (HVSFS). Journal of Materials Science: Materials in Medicine. 2011; 22 :1303-1319 - 102.
Cañas E, Orts MJ, Boccaccini AR, et al. Microstructural and in vitro characterization of 45S5 bioactive glass coatings deposited by solution precursor plasma spraying (SPPS). Surface & Coatings Technology. 2019; 371 :151-160 - 103.
Sergi R, Bellucci D, Cannillo V. A comprehensive review of bioactive glass coatings: State of the art, challenges and future perspectives. Coatings. 2020; 10 :757 - 104.
Garrido B, Dosta S, Cano IG. Bioactive glass coatings obtained by thermal spray: Current status and future challenges. Boletín de la Sociedad Española de Cerámica y Vidrio. 2022; 61 :516-530