A list of the different low-carbon steel-grade tubulars and their composition (API specification 5CT).
Abstract
The focus of this chapter will be on corrosion during downhole oil and gas treatments such as matrix acidizing, inorganic scale removal, and mud filter cake removal. The chapter will start by introducing the nature of oil and gas treatments, defining the types of corrosion, sharing the type of metallurgy, and the effect of alloying for tubulars used in the oilfield. After that, corrosive elements such as gases will be discussed. Common corrosion mechanisms and prevention methods that include the use of corrosion inhibitors and intensifiers will be shared. Additionally, the chapter will mention the passing criteria in the industry and the most notable chemical incompatibilities encountered. Moreover, the toxicity of these corrosion inhibitors will be touched on briefly and the chapter will end with environmentally friendly options for corrosion inhibition in the oilfield.
Keywords
- matrix acidizing
- inorganic scale removal
- filtercake
- low-carbon steel
- corrosion resistant alloy
- corrosion inhibitor intensifier
- strong acid
- high temperature
- toxicity
- environmentally friendly
1. Introduction
Corrosion is a worldwide problem that concerns the stability of bridges, buildings, equipment, and any metallic structures. More notably, the oil and gas industry incurs a cost of corrosion approximating $1.372 billion dollars annually [1]. Corrosion is witnessed in several sectors of the oil and gas industry from downhole tubulars to surface pipelines and processing facilities. It poses an integrity risk that compromises well-control capabilities, especially at high pressures threatening the environment and human safety.
The oil and gas industry deploys a variety of treatments that utilize acids to enhance production rates. Matrix acidizing refers to a stimulation treatment that involves pumping acidic fluids in the wellbore to react with the formation rock. These fluids are typically pumped at high pressures just below the formation fracture pressure. It aims to generate deep wormholes to bypass the near wellbore damage that exists due to the drilling process, Figure 1.
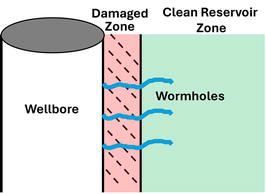
Figure 1.
Matrix acidizing job resulting in wormhole creation and bypassing near wellbore formation damage.
Some commonly used acids in these treatments include inorganic acids such as hydrochloric acid (HCl) for carbonate formations. Sandstone formations undergo a slightly different process. Commonly, a combination of Hydrofluoric acid (HF) and Hydrochloric acid is used at a ratio of 4:1 (12 wt.% HCl: 3 wt.% HF), where the acid soaks the near wellbore area with no intent to create wormholes. Alternatively, organic acids such as acetic acid, lactic acid, formic acid, and aminopolycarboxylic acids can be used for both formations [2, 3].
Inorganic scale precipitations occur when incompatible water sources are mixed, and it is more notable at locations where pressure and temperature significantly fluctuate. These scales are commonly encountered at chokes, twists, and turns of surface tubulars, downhole at the intake of electrical submersible pumps, or at the top of the wellbore where the gases such as carbon dioxide (CO2) are liberated. Inorganic scale can accumulate in the wellbore resulting in a production loss. Another form of oil and gas treatment is the removal of inorganic scale precipitations which include carbonate, sulfate, or sulfide scales. Carbonate scales require the use of HCl, while sulfate and sulfide scales can be dealt with using aminopolycarboxylic acids or combinations of inorganic and organic acids [4, 5].
The main purpose of drilling fluid is to maintain well control and stability while drilling. Additionally, drilling fluids are expected to lift formation cuttings efficiently through the wellbore and cool down the drilling bit. These fluids also generate a thin and impermeable layer which is referred to as “filtercake”. The generated filtercake is formed due to a pressure difference between the wellbore and the formation. It serves as a protective layer and creates a barrier that prevents the formation of fluids from flowing into the wellbore. After completing the drilling operation and prior to initiating production a filtercake removal treatment is generally desired. The filtercake is composed of polymers and insoluble particulates used as weighting material. Some applied examples of these particles include calcium carbonate, iron oxide, manganese tetroxide, or barium sulfate. It is essential to use the appropriate acid to dissolve them which in turn removes the filtercake more efficiently. It is also necessary to remove the filtercake gradually and uniformly to avoid well-control issues [6]. This requires exposing the tubular to an acidic treatment for a longer duration of time.
The use of acidic fluids is a recurring solution to many problems in the oil and gas industry. However, due to the use of acids, corrosion to downhole equipment and tubulars is a major cause for concern.
2. Types of corrosion
Corrosion in simple terms is defined as the interaction between the metal and its environment which results in the eventual loss of the material. Corrosion can be classified into 8 different categories [7]: Uniform corrosion, pitting corrosion, intergranular corrosion, galvanic corrosion, crevice corrosion, erosion corrosion, selective leaching, and stress-corrosion cracking. They will be introduced briefly below:
2.1 Uniform corrosion
This is an ideal form of corrosion, where the electrochemical process results in an even removal of the material, Figure 2.
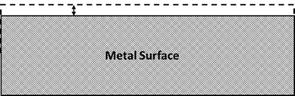
Figure 2.
Uniform corrosion of metal surface.
An electrochemical cell consists of an anode, a cathode, an aqueous medium, and an electronic or metallic pathway where electrons can pass through, Figure 3.
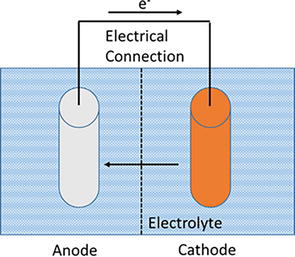
Figure 3.
Uniform corrosion showing the components of an electrochemical cell.
Uniform corrosion is considered the simplest type of corrosion and is assumed to be the case when conducting corrosion laboratory tests. However, the process of uniform corrosion is seldom seen in real-life applications as corrosion occurs in combinations due to a variety of environmental and internal factors such as the alloying process, temperature, and grain structure. These factors can produce several anodic and cathodic sites in the metal. The cathodic sites are responsible for the liberation of hydrogen while the anodic sites are involved in the dissolution of the metal. This in turn results in combinations of corrosion processes which signifies the importance of investigating localized corrosion problems.
2.2 Pitting corrosion
The process of repetitive removal of metal from the anodic site starts to generate deep holes or “pits”. These pits represent depressions in the metal surface, and they can vary in size from microscopic to clearly visible. Pits are considered highly corrosive areas and can vary in depth and width throughout the corrosion process. An illustration of different pitting corrosions can be seen in Figure 4.
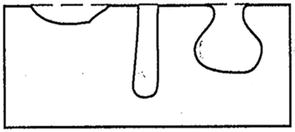
Figure 4.
A simplified schematic showing examples of pitting corrosion with changes in the depth and width of the pit [
Pits can also get complicated and hard to discover due to the formation of corrosion products that can cover the pit opening, keeping them hidden from sight while corrosion continues progressing within it. This form of corrosion requires rigorous monitoring as it can be hard to predict. Pitting corrosion often occurs due to having an excess of certain anions that can be monitored, controlled, and avoided. Common anion examples faced in the oilfield include chloride or bromide which are ordinarily seen in matrix acidizing, filtercake removal, and inorganic scale removal treatment recipes.
2.3 Crevice corrosion
Crevice corrosion is very similar to pitting corrosion since they both cause weaknesses and develop due to localized corrosion mechanisms. However, they differ in location. Pitting can occur anywhere on the surface of the metal. Alternatively, crevice corrosion is found between gaps in the metal. These gaps result in a highly localized and corrosive environment. Crevice corrosion is still influenced by similar anions known to be troublesome in pitting corrosion. However, this type of corrosion is much more easily dealt with as the location is determined and the gap can be amended preventing further damage to the metal.
2.4 Intergranular corrosion
Intergranular corrosion is a form of corrosion that occurs between the grain boundaries in the metal. It happens due to a variety of reasons that include segregation of impurities, precipitation of carbide at the boundary, or the removal of passivation elements. All these factors can further result in localized galvanic or the formation of depletion zones which increase the metal’s susceptibility to corrosion stress cracking.
2.5 Corrosion stress cracking and hydrogen embrittlement
This corrosion occurs due to hydrogen’s small size which can diffuse into the metal matrix through imperfections such as pits, crevices, or intergranular corrosion sites. As pressure decreases, hydrogen significantly increases in volume imposing high stresses on the metal structure that results in weakening it. This increases its susceptibility to cracking and mechanical failure. The source of hydrogen can emanate from acidic-produced gases such as hydrogen sulfide (H2S) or from the use of acidic mediums in oilfield treatments. This phenomenon is commonly seen in pits as stress can accumulate and crack the metal. Sulfide is also known to poison H2 by preventing the recombination of hydrogen atoms and that accelerates the corrosion process.
2.6 Galvanic corrosion
Galvanic corrosion occurs when two dissimilar metals are coupled and immersed in a highly conductive solution. It is also known as bi-metallic corrosion and represents a process where one metal is corroded in preference to the other. The higher anodic metal will undergo a localized corrosion attack near the boundary where they are coupled. This is common in the oil and gas industry due to transporting seawater, produced water, and spent acid treatments through surface pipelines. An illustration is seen in Figure 5.
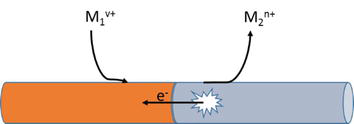
Figure 5.
A representation of galvanic corrosion in two dissimilar metals.
2.7 Erosion corrosion
This form of corrosion involves the physical removal of metal layers. Erosion corrosion occurs due to high-velocity turbulent flow of corrosive fluids and can be exacerbated in the presence of sand or particulates such as inorganic scale matter. It is unique compared to other forms of corrosion due to its ability to physically remove the deposited corrosion products. This exposes the fresh metal and results in an accelerated corrosion process. Erosion corrosion is more common when flowing back wells after acidic treatments in the oil and gas industry [8].
2.8 Selective leaching corrosion
Selective leaching is also known as “de-alloying” and is defined as the process where certain elements in the metal alloy are being selectively leached. This occurs in elements that are more anodic than others. An example would be the removal of zinc and being selectively leached instead of copper resulting in a more compromised copper material that will undergo metal failure easily [8].
3. Metallurgy
The most common metal used for wellbore tubulars, completions, and surface pipelines in the oil and gas industry is made of low-carbon steel (LCS). As the name implies, low-carbon steels are a mixture of carbon and steel that contains a low-carbon content (<0.4 wt.%). The two other forms of carbon steel are medium carbon steel (0.4–0.6 wt.%), and high carbon steel (0.6–1.5 wt.%).
3.1 Low-carbon seel
Low-carbon steel tubulars are mainly composed of manganese and iron with impurity content. Examples of low-carbon steel tubulars used in the oilfield include J-55, L-80, T-95, P-110, and Q-125. The letters represent the grade of LCS which can be achieved through a variety of post processing techniques such as quenching or tempering. The numbers represent the yield strength rating, where the higher number amounts to a higher yield strength tolerance. Although all LCS tubulars maintain a comparable composition of elements they still differ in mechanical and chemical properties including H2S gas tolerance and corrosivity to acids. These differences are attributed to the changes in element impurity content, the size, and the distribution of the grain microstructure resulting from post processing.
For example, J-55, a normal grade LCS tubular represents the simplest type of low-carbon steel as it does not require any special post treatments to achieve the desired mechanical properties. This type of steel can be prepared by cooling it naturally (leaving it to stand). Consequently, it achieves a very large grain microstructure. Alternatively, LCS such as P-110, which in most cases utilizes the same raw steel material as J-55, presents higher yield strength due to changing the lattice microstructure of the metal through post processing techniques of tempering and quenching cycles. This generates a metal with highly desired mechanical properties and would lead to a dramatic price increase resulting in P-110 being a more costly type of LCS in comparison to J-55.
The final steel properties can also be limited by the quality of the raw material and that can determine the ability to pass standard steel qualifications to deem it acceptable in the oil and gas industry. Generating the highly rated Q-125 can require multiple repetitive cycles of tempering and quenching in addition to using the best quality raw material available.
3.2 Corrosion resistant alloys
Another class of metal used is Corrosion Resistance Alloys (CRA). They are higher in cost and are distinguished by the higher concentration of elements such as chrome, molybdenum, and nickel. These CRAs can be further divided into 2 categories: High chrome content (e.g., Cr13 and Superchrome) and specialty alloys. High chrome tubulars generate a passive layer of Cr2O3, which provides a shiny mirror-like appearance along with a resistance to oxidation and CO2 corrosion. The Cr2O3 protective layer is dissolved in the presence of strong acids such as HCl. Moreover, it is easily damaged and compromised in the presence of H2S. For that reason, extreme care must be taken when conducting acidic treatments in the presence of such tubulars to maintain their integrity, functionality, and prevent additional maintenance-related expenses.
Specialty alloys can include nickel-based metals such as Incoloy or Hastelloy. These types are used in environments that are considered very corrosive for low-carbon steel and high chrome content metals. The petroleum industry lab reactors are typically manufactured with this material as they can handle a variety of acids used in oil and gas treatments. Nonetheless, it carries a very hefty price that prevents wide use in surface and downhole well tubulars. A general comparison of common LCS and CRA tubular compositions is seen in Table 1.
Grade | Type | C | Mn | Mo | Cr | Nb | Ni | Cu | P | S | Si |
---|---|---|---|---|---|---|---|---|---|---|---|
Max | Max | Max | Max | Max | Max | Max | Max | Max | Max | ||
H40 | — | — | — | — | — | — | — | — | 0.03 | 0.03 | — |
J55 | — | — | — | — | — | — | — | — | 0.03 | 0.03 | — |
K55 | — | — | — | — | — | — | — | — | 0.03 | 0.03 | — |
N80 | 1 | — | — | — | — | — | — | — | 0.03 | 0.03 | — |
N80 | Q | — | — | — | — | — | — | — | 0.03 | 0.03 | — |
R95 | — | 0.45c | 1.90 | — | — | — | — | — | 0.03 | 0.03 | 0.45 |
L80 | 1 | 0.43a | 1.90 | — | 1.50 | — | 0.25 | 0.35 | 0.03 | 0.03 | 0.45 |
L80 | 3Cr | 0.30 | 1.20 | — | 2.5–3.9 | 0.30 | 0.25 | 0.35 | 0.02 | 0.01 | 0.45 |
L80 | 9Cr | 0.15 | 0.3–0.6 | 0.9–1.1 | 8.0–10.0 | — | 0.50 | 0.25 | 0.02 | 0.01 | 1.00 |
L80 | 13Cr | 0.15–0.22 | 0.25–1 | — | 12.0–14.0 | — | 0.50 | 0.25 | 0.02 | 0.01 | 1.00 |
C90 | — | 0.35 | 1.20 | 0.25b-0.85 | 1.50 | — | 0.99 | — | 0.02 | 0.01 | — |
T95 | — | 0.35 | 1.20 | 0.25d-0.85 | 0.4-1.5 | — | 0.99 | — | 0.02 | 0.01 | — |
C110 | — | 0.35 | 1.20 | 0.25–1 | 0.4–1.5 | — | 0.99 | — | 0.02 | 0.005 | — |
P110 | — | — | — | — | — | — | — | — | 0.030e | 0.030e | — |
Q125 | — | 0.35 | 1.35 | 0.85 | 1.50 | — | — | — | 0.02 | 0.01 | — |
Table 1.
The carbon content for L80 may be increased up to 0.50% max if the product is oil-quenched or polymer-quenched.
The molybdenum content for C90 Type 1 has no minimum tolerance if the wall thickness is less than 0.700 in.
The carbon content for R95 may be increased up to 0.55% max if the product is oil-quenched.
The molybdenum content for T95 Type 1 may be decreased to 0.15% min if the wall thickness is less than 0.700 in.
For EW Grade P110, the phosphorus content shall be 0.020% max and the sulfur content 0.010% max.
3.3 Effects of alloying
Certain elements are added to the metal composition at specific ratios to provide improvements to both its mechanical properties and corrosion tolerance. This is critical for well cases that produce large volumes of corrosive gases such as H2S and CO2 to maintain their integrity throughout the hydrocarbon production lifetime. Examples of these elements and a brief description of their provided properties can be seen below:
Carbon (C): one of the major components in steel. The purpose of adding this element is to form martensite and carbides that can contribute to the hardness of steel. Additionally, it provides more resistance to wear. However, excessive carbon content can produce more brittle steel with a reduced toughness profile and can highly influence the electrochemical corrosion process. A high content of carbon can increase pearlitic and reduce ferritic phases in the steel. The higher the pearlitic phase the lower the anodic corrosion. On the other hand, excessive carbon content can increase the chance of converting the pearlite into cementite which would accelerate corrosion rate [9]. For higher H2S-producing wells, the low-carbon content can help significantly in mitigating cases of sulfide stress cracking (SSC) [10]. Lastly, the content of carbon in the steel is also often associated with the naming of the steel as mentioned earlier.
Manganese (Mn): this element is typically added to remove oxide and sulfur impurities. It also provides added benefits by forming finely divided grain microstructures, which increases strength and reduces the ductile transition temperature in steel [11]. One of the main benefits of adding manganese is an increase in the stability of steel during cooling phase. This occurs through reducing the shock experienced in the microstructure. Manganese works well in steel manufacturing and provides a cheaper replacement for nickel in the mixture composition.
Nickel (Ni): works similarly to manganese; however, it is more expensive in comparison. Nickel content can improve hardness by creating finer grain microstructures and by creating more stability when quenching the steel. Nickel can also improve strength and oxidative resistance while reducing ductility [11]. It is also known that the carbon content in the steel can influence the performance of nickel in the alloy.
Chromium (Cr): this element is added to the steel composition to add oxidation resistance. This property occurs due to the formation of a Cr2O3 layer which can enhance steel tolerance to oxygen and CO2 environments. When chrome content is at 10.5 wt.% the alloy is given the name stainless steel. Having chrome in the alloy can also improve the hardness and wear tolerance.
Molybdenum (Mo): used to prevent cases of localized corrosion through the molybdate anion [12]. Molybdenum is also added to increase tensile strength and hardness at high temperatures. To achieve the desired performance, it is seldom used alone and is often combined with chrome and nickel.
Vanadium (V): Enhances the steel strength and toughness by controlling grain growth during heat-treating processes. Additionally, it works as an oxygen scavenger and can provide a secondary hardening effect.
Copper (Cu): provides resistance to atmospheric corrosion. Moreover, it can provide pitting and corrosion resistance against certain acids when incorporated at concentrations above 0.2 wt.% [13].
Silicon (Si): added for its ability to work as a deoxidizer providing protection from oxidation. It can also add strength, hardness, and toughness to the steel [11].
Phosphorous (P): this element normally represents impurity; however, it can be meticulously added to the steel mixture to increase strength, machinability, and corrosion resistance [14]. More specifically, the addition of phosphorous at concentrations above 0.4 wt.% may lead to a decline in weldability and toughness. For that reason, the addition of this element must be constrained.
4. Corrosive elements
The high energy state of the tubular metals provides a corrosive incubator when interacting with its surrounding environment through mechanical, chemical, and electrochemical means. Elements favoring the energy loss from excess electrons exacerbate the corrosion effect. These elements which create an aggressive corrosive environment are water, pH, and gases such as H2S or CO2 especially under high temperature and pressure [15]. The presence of each element plays a significant role in corrosion and is highly influenced by flow dynamics.
An environment that contains hydrogen sulfide is defined as “sour”, whereas the absence of it defines it as “sweet”. Hydrogen sulfide is a gas that generates a weak acid in water from the dissociation leading to the generation of hydrogen bisulfide (HS-). This disassociated H2S gas reacts with metal equipment creating corrosion products of iron sulfides and hydrogen, Figure 6. These molecules result in a hydrogen embrittlement phenomenon known as sulfide stress cracking (SSC) when exposed to the metal. An increase in SSC can be observed when the internal structure is exposed to large amounts of the molecule increasing stress embrittlement. The results of H2S corrosion on the tubulars are complex [16]. While SSC is a major concern, several studies favor the formation of iron sulfide from the presence of H2S as it mitigates further corrosion. These positive studies suggest a two-layer solution of solid-state diffusion barrier from an inner mackinawite film (iron sulfide) and a porous outer flaky fragmenting iron sulfide layer. This two-layer solution is widely accepted to be a possible product and limitation of corrosion rate from H2S gas [17]. The mechanism for this two-state barrier formation has yet to be confirmed and is still being researched. A variety of these studies suggest that multiple forms of iron sulfide are present based on water chemistry, H2S exposure, and thermodynamic stability [18]. While the effects of H2S corrosion products are not always negative, the presence of H2S in formation fluid hydrocarbon accounts for almost 40% of the annual corrosion cost.
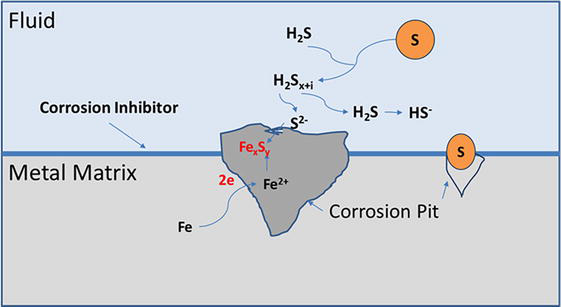
Figure 6.
Hydrogen sulfide disassociation and corrosion with metal even in the presence of some corrosion inhibitors [
The next element, CO2, is a gas that also generates a weak acid when dissolved in water in the form of carbonic acid (H2CO3). This acid lowers the pH of the fluid resulting in corrosion and pitting of steel. The effect of CO2 corrosion is highly dependent on several factors including partial pressure, temperature, presence of H2S, oxygen, water chemistry, pH, and hydrodynamic conditions [20]. The sweet corrosion from CO2 is generally increased under high partial pressure and temperature. This reaction may be reduced based on the other mentioned factors.
Certain amounts of H2S have been proven to reduce CO2 and overall corrosion in the system. The presence of H2S in sufficient amounts at the correct conditions can produce a coating of iron sulfide on the tubular. This layer can prevent corrosion due to CO2. It is widely accepted in the oil and gas industry that the presence of H2S with CO2 is more desired than a purely sweet environment. This inhibition phenomena can however be overpowered. If CO2 partial pressure and flow velocity exceed protection capabilities, an increase in localized corrosion and pitting is observed [17, 21, 22]. In general, CO2 corrosion is dealt with by using high chrome-based tubulars. A Chrome composition of over 10% is required to be effective in sweet environments.
Another element is water, which is an essential element for all corrosion including those caused by the gases mentioned. Water chemistry plays a major role in corrosion and its chemistry can either increase or mitigate corrosion. Generally, a decrease in water pH results in higher corrosion. The acidity coupled with dissolution of different molecules leads to a higher corrosion of the tubulars. A higher salt concentration in water is typically more corrosive. The high chloride content results in a more aggressive fluid reaction with the steel tubular. While majority of studies have shown that salt increase leads to more localized corrosion, several show that high salt content in the presence of CO2 reduces corrosion [23].
5. Corrosion in the oil and gas industry
The use of strong acids is favored in most of the oil and gas treatments to improve formation productivity. Additionally, the presence of high concentrations of chloride ions is common due to the use of hydrochloric acid (HCl). Hydrochloric acid is the preferred choice because it’s a strong acid that has a high dissolution capacity toward carbonate minerals (calcite/dolomite carbonate formations, carbonate inorganic scales, carbonate mud weighting material). Moreover, HCl is relatively cheap, and the reaction products are highly soluble preventing further damage to the formation [24].
The existence of chloride ions in solution can result in a combination of corrosion types that include uniform, crevice, and pitting corrosion [25]. Furthermore, the presence of H2S can raise concerns about sulfide stress cracking.
Corrosion resulting from the use of strong acids such as HCl would initially dissolve the oxide layer on the metal, as seen in
Fe (III) oxide:
Cr (III) oxide:
Oxide layers are formed due to exposing the base metal to air undergoing oxidation. The oxide layer protects the bulk metal from exposure to the environment and the thickness of this layer will depend on a variety of factors such as the temperature it was exposed to and duration of exposure. Experiencing a higher temperature and exposure time can result in much thicker oxide layers [26].
Once the oxide layers are dissolved, an electrochemical reaction between H+ and the bulk metal occurs. Imperfections are bound to exist in the metal and due to these imperfections, the metal can develop localized cathodic and anodic sites which would facilitate the corrosion process.
Reduction of H+ ions occur at the cathodic sites through the donation of a pair of electrons from the iron atoms at the anodic site, Eqs. (3)–(4) and the overall electrochemical reaction can be seen in Eq. (5):
Cathodic reaction:
Anodic reaction:
Overall electrochemical reaction:
The presence of certain compounds such as cementite (a form of carbide) can also influence the corrosion process. It works on accelerating corrosion by providing a desirable lower overpotential cathodic site to form hydrogen [27].
6. Corrosion inhibitors
The introduction of the various elements in the metal in addition to processing techniques, results in diverse mechanical and chemical properties even within the same manufacturer’s steel and will create alloy families with various grades.
After installing the tubulars, wells are expected to continue production for tens of years. Additionally, although some tubular replacement is possible, the process itself is difficult, dangerous (blowouts, production of toxic gases, etc..), and costly. Consequently, it becomes important to protect pipelines and downhole tubulars from corrosion, especially during treatments where strong acids and corrosive fluids are encountered.
To contest this challenge, the industry employs a variety of methods that include using acid-resistant steel, coating the steel, cathodic/anodic protection methods, and the use of mixed corrosion inhibitor chemicals.
These methods can be applied in combination to protect the pipelines due to the harsh environments presented in the oil field. Moreover, the industry is continuously working on replacing the use of strong acids such as HCl with a milder and yet more effective acidizing fluid.
It is necessary to note at this point that corrosion will still occur no matter what protection combination is considered. However, the goal of employing these methods is to reduce corrosion rates. This will reduce the cost of repairing the tubulars and will also provide a much safer working environment.
While the use of acid-resistant tubulars sounds attractive, it is not economically feasible to replace all tubulars. The industry does, however, install sections of tubulars with Hastelloy grade metal in high-temperature wells that face significant H2S, and CO2 content when producing hydrocarbons [28]. These cases still present concerns regarding galvanic corrosion that can arise at the contact point between the different steel grades.
The use of alternative acids to HCl has also been considered and applied in industry. Such examples include the use of weaker organic acids such as acetic, formic, or even aminopolycarboxylic acids.
Organic acids have a lower reaction rate, resulting in a favorable lower corrosion rate with the steel. At high-temperature conditions, the generated wormholes are deep, exhibit limited branching, and can cross the damage zone with minimal acid volume consumption. Comparatively, the use of HCl at high-temperature conditions results in aggressive reaction rates with both the steel and formation rock [29]. This will damage the tubulars and result in “face dissolution” toward the formation rock, thus, compromising its ability to generate wormholes that can bypass the damage zones downhole [30]. Furthermore, it has the potential to cause sludge and asphaltene precipitation if mixed with an incompatible crude [31]. On the other hand, organic acids have a lower tendency to form sludge and emulsion when mixed with crude oil. However, organic acids such as acetic, formic, and citric acid will precipitate when exposed to higher calcium concentrations, and aminopolycarboxylic acids tend to be more expensive in comparison to HCl [24].
Nonetheless, the use of HCl along with corrosion inhibitors is still the preferred method of protection. Corrosion inhibitors must be incorporated in every treatment that uses acid. Corrosion inhibitors exist in liquid form such as quaternary ammonium compounds, or in gas form such as carbon monoxide. They can further be divided into three categories depending on their protection mechanism: Cathodic, anodic, or mixed inhibitors [32].
When conducting a matrix acidizing job in the oilfield, organic corrosion inhibitors are the most used and they act as a mixed inhibitor for both the anodic and cathodic sites on the steel. These organic molecules contain polar elements such as Nitrogen, phosphorus, oxygen, or sulfur. The polar elements along with having hydrophobic sites or electron donating benzene rings allow it to adsorb onto the steel.
When steel is exposed to fluids containing HCl, the chlorine ion lines up on the outer steel surface providing it with a negative charge. The most utilized corrosion inhibitors are cationic to provide strong attraction to the tubular. Some examples of these corrosion inhibitors include quaternary ammonium compounds. Other examples include imidazolines and acetylenic alcohol compounds [33, 34, 35, 36, 37]. Figure 7 illustrates the structural formulas of corrosion inhibitors used in the oilfield.
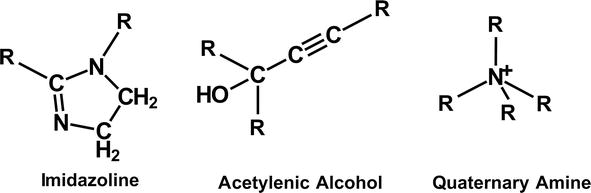
Figure 7.
Common oil and gas corrosion inhibitors.
Commercial corrosion inhibitors contain a mixture of these compounds, along with solvents and salts that enhance adsorption and the formation of the protective film on the steel.
In cases where organic acids are used, chlorine ions are absent, and a different inhibitor chemistry is required. Corrosion inhibitors containing sulfur have shown success with organic acids. Some examples of sulfur-containing corrosion inhibitors are thiourea-based chemistries.
7. Corrosion inhibitor intensifiers
When strong acids such as HCl are used at high-temperature conditions (>200°F), the reaction rate is increased significantly, and it becomes difficult to control the acid interaction with the steel or the formation rock. Retarding or slowing down HCl reaction rate can help in this regard, and it can be economically achieved by increasing its viscosity (addition of polymers) or by emulsifying it in diesel as seen in Figure 8.
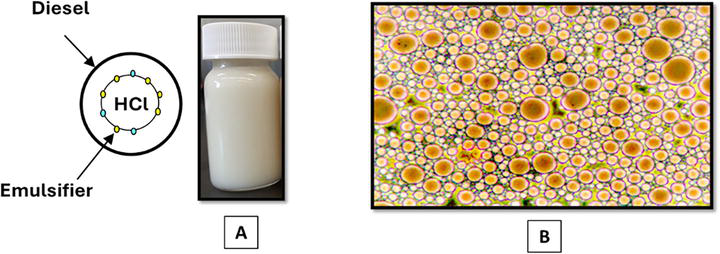
Figure 8.
(A) Emulsified acid exhibits a milky and viscous appearance, (B) emulsified acid under the microscope showing emulsion droplets.
These two methods slow down the interactions with the rock successfully and maintain a successful wormhole propagation profile, however, the reactions with the steel are still not controlled and effectiveness of corrosion inhibitor molecules suffers beyond its maximum operating temperature. Accordingly, corrosion inhibitor intensifiers must be used.
Corrosion inhibitor intensifiers are compounds that can be added to the corrosion inhibitor to augment its performance at high-temperature conditions. Some examples of these inhibitor intensifiers include the use of simple salts such as potassium iodide, antimony chloride, copper salts, and formamides [38, 39, 40]. Copper salts would result in a redox reaction (Eq. 6) and the formation of a copper layer which has low reactivity and resistance to acid, Figure 9.
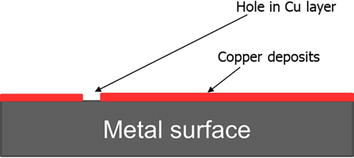
Figure 9.
A simplified representation of cupper working as a corrosion inhibitor intensifier.
On the other hand, Iodine would line up on the metal providing a stronger charge for the corrosion inhibitor to adsorb on the metal [41]. This can be seen in Figure 10.
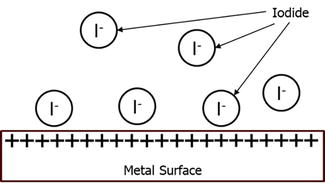
Figure 10.
Potassium iodide lining onto the surface of the metal.
The use of antimony and copper salts is limited in several countries due to environmental concerns. Alternative approaches include the use of organic acids as intensifiers. Formic acid is an excellent corrosion inhibitor intensifier where it relies on its ability to degrade in the presence of HCl and the reaction is catalyzed by metal surfaces to produce carbon monoxide. This phenomenon has been studied thoroughly in the past [42, 43, 44], Eqs. 7–10. Linear bonds are formed first and as the metal surface becomes more saturated, bridging bonds are formed between carbon monoxide and the metal resulting in the blocking of multiple active sites by a single carbon monoxide molecule [45]. An illustration can be seen in Figure 11.
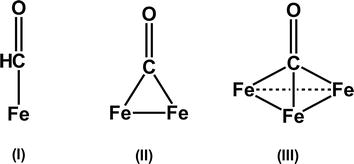
Figure 11.
Linear and bridged bonding of carbon monoxide on the steel surface.
The secondary reaction of CO on the steel surface involves further decomposition of CO which leads to the formation of a layer of metal carbides [46, 47]. While iron carbides can promote corrosion by increasing the H2 overpotential, they can also reduce corrosion rates if their particle size is large enough [27].
Intensifiers can also be combined to provide a more economical solution than the use of a single intensifier at high concentrations [27]. It is wise to note that the intensifiers work to support the main corrosion inhibitor molecule and chemical compatibility should be accounted for to be able to handle harsh oilfield conditions [48, 49, 50].
8. Standard and requirements
8.1 Corrosion inhibitor passing criteria
Corrosion inhibitors are required to be tested for reliability based on the implementation environment. These inhibitors are utilized in different sectors from drilling to production and processing. It is necessary to note that while corrosion is a product of different operations, a rigid criterion is only set industry-wide for the most corrosive of operations which include acidizing treatments. Even though an acid treatment can last up to 2 hours, the lab testing criteria for the passing requirements is set for a 6-hour corrosion immersion test using a reactor.
To understand the corrosiveness of any acidizing fluid, tests are often done using the desired formulation and metal coupons made from similar metal to that of downhole tubulars. The test methods follow the ASTM G31 standard for corrosion immersion tests. If the corrosion rate of the tested solution exceeds 0.05 lb/ft2 for low-carbon steel or 0.03 lb/ft2 for corrosion-resistant alloys, it is too corrosive to be used [51, 52]. However, this standard may be lowered in lieu of other factors such as the cost of the metal used or ease of replacement and material transportation [53, 54]. For example, the corrosion rate allowed for coiled tubing operations is less than 0.02 lb/ft2. Pitting of the coupon is also taken into consideration when analyzing the results of the test as pits can severely undermine the structural integrity of the metal. Considerable work has also been conducted to evaluate high-temperature corrosion environments, and the passing criteria may be lowered due to the aggressive reactions at higher temperatures [55].
8.2 Corrosion inhibitor compatibility
Acid treatments in the oilfield consist of a variety of chemicals such as surfactants, polymers, H2S scavengers, Scale inhibitors, emulsifiers/demulsifiers, and anti-sludge agents. Due to the diverse chemical families, functional groups, and charges involved, it is crucial to test for chemical compatibility and ensure the functionality of each additive. Corrosion inhibitors are known for incompatibility with certain additive combinations and have been an increasingly growing area of research throughout the years.
The first common incompatibility is seen between viscoelastic surfactants (VES) and corrosion inhibitors. This incompatibility prevents either chemical from functioning properly. Viscoelastic surfactants are utilized in acid treatments to generate a diversion effect by increasing the viscosity of the fluid significantly. This improved the distribution of acid in the treatment zones. However, the presence of corrosion inhibitors has always caused a failure to build the intended viscosity. This could be attributed to the surfactant-like behavior seen in corrosion inhibitors which consequently distributes the shape of the VES micelles formed [56].
The second incompatibility is noted when scale inhibitors are utilized. Corrosion inhibitors exhibit a positive charge that allows them to adsorb onto the steel surface in the presence of strong acids such as HCl. On the contrary, scale inhibitors are typically composed of acrylic acid and include similar negatively charged side groups. The existence of both chemicals in the same pumped fluid can cause an attraction between the two molecules and possibly lead to precipitation. This would result in a failure to perform the intended job [57].
The third incompatibility exists when emulsifiers/demulsifiers are used. This incompatibility is very common in treatments that include the pumping of emulsified acids stages. The interactions between certain corrosion inhibitors and emulsifying surfactants can result in failure to form a stable emulsion. In many cases the separation is instant, and this would jeopardize the treatment as the reaction rate is significantly increased if the acid does not maintain an emulsified form, Figure 12. In opposite scenarios, the corrosion inhibitor can form a very stable emulsion causing formation damage and difficulties for the surface refineries and treatment facilities when dealing with flow back returns [58].
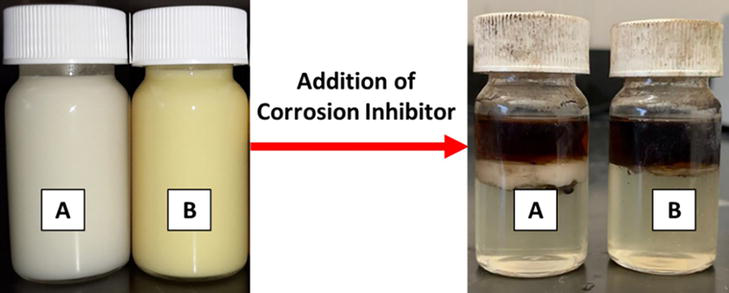
Figure 12.
Emulsified acid compatibility with corrosion inhibitor.
Another incompatibility is the interaction with iron contamination. Iron exists in both ferrous and ferric forms in the industry and the source of iron can range from minerals, inorganic scale, tubulars, pumps and equipment, formation water, or even the pumped acid itself can contain iron. The interaction with iron contaminants is undesirable as it prevents the corrosion inhibitor from adsorbing to the intended target [59].
The last incompatibility occurs in the presence of H2S. Corrosion inhibitors are not able to perform a successful inhibition because H2S can bypass the corrosion inhibitor corroding the steel and resulting in the formation of iron sulfide. The best way to deal with this concern is to use H2S scavengers, however, these scavengers might react with the corrosion inhibitor if not chemically compatible and they can also negatively influence other additives such as demulsifiers [19, 60].
Even though the corrosion inhibitor chemical might have passed the testing requirements in lab conditions, transferring these molecules to field conditions may cause failures due to the plethora of negative interactions that can occur with incompatible chemistries. A thorough compatibility assessment with a variety of additives and contaminants is necessary for applications in the oilfield.
9. Environmentally friendly corrosion inhibitors
Corrosion inhibitors are one of the most toxic and environmentally damaging chemicals encountered in oil and gas treatments. The material safety data sheets (MSDS) show that these compounds are rated as toxic and damaging to the environment and human body. They cause harm by inhalation, when in contact with skin and if swallowed. Additionally, they are harmful to aquatic organisms and may cause adverse effects in aquatic environments.
The corrosion inhibition adsorption functionality is initiated due to the presence of polar elements that can share electrons with the steel. The ionic charge of this molecule also plays a role in this ability to stick. Compared to conventional corrosion inhibitors, there are many environmentally friendly, edible, and non-toxic alternatives that provide similar characteristics while remaining safe to handle.
Environmentally friendly corrosion inhibitor compounds can be derived from a variety of plant parts such as seeds, stems, leaves, flowers, fruits, and roots [61, 62, 63, 64, 65, 66, 67]. Some examples include inhibitors made from cinnamon, garlic, thyme, rosemary, mint, and turmeric. Other recent research has also investigated reducing the accumulation of waste by utilizing what is considered trash to extract effective corrosion inhibitors for the oilfield [68, 69, 70, 71, 72]. Their efficiency, ability to handle high-temperature conditions, compatibility with other additives, and functionality in high concentrations of acid used in the oilfield an important area of research that is ongoing up to this day.
10. Conclusions
This chapter has presented a summarized version of corrosion in the oilfield. It initially defined the different treatments that are a corrosion concern. It then discussed the different types of corrosion and noted that corrosion in the industry is not a single form but typically a combination of different corrosion mechanisms that occur due to variations in acid type, steel type, and the presence of critical corrosive elements. The chapter then discussed the different types of steel encountered in the oilfield along with the influence of elements in the alloying process. The standard for the different steel types was also shared along with the minimum requirements to approve a corrosion inhibitor for the oilfield. The chapter then discussed the corrosion process where strong acids are used for matrix acidizing, filter cake removal, and inorganic scale removal jobs. The main characteristics of corrosion inhibitors and intensifiers were shared showing some commonly applied chemical families. The most important portion of this chapter was the corrosion inhibitor compatibility as this is an issue that is significantly overlooked. The pumped treatment consists of a variety of additives that require careful consideration when developing a corrosion inhibitor package for field applications. Lastly, the chapter went through the toxicity and examples of work done in the environmental aspect of corrosion inhibitors.
Nomenclature
carbon dioxide | |
corrosion resistant alloy | |
hydrogen sulfide | |
hydrochloric acid | |
hydrofluoric acid | |
low-carbon steel | |
National Association of Corrosion Engineers | |
sulfide stress cracking |
References
- 1.
Popoola L, Grema A, Latinwo G, et al. Corrosion problems during oil and gas production and its mitigation. International Journal of Industrial Chemistry. 2013; 4 (1):1-15. DOI: 10.1186/2228-5547-4-35 - 2.
Hassan A, Mahmoud M, Bageri B, et al. Applications of chelating agents in the upstream oil and gas industry: A review. Energy & Fuels. 2020; 34 (12):15593-15613. DOI: 10.1021/acs.energyfuels.0c03279 - 3.
Almubarak T, Ng J, Ramanathan R, et al. Chelating agents for oilfield stimulation: Lessons learned and future outlook. Journal of Petroleum Science and Engineering. 2021; 205 :108832. DOI: 10.1016/j.petrol.2021.108832 - 4.
Almubarak T, Ng JH, Nasr-El-Din H. Oilfield scale removal by chelating agents: An aminopolycarboxylic acids review. In: Presented at the SPE Western Regional Meeting, Bakersfield, California, April 23–27, 2017. Texas, USA: OnePetro; 2017. DOI: 10.2118/185636-MS - 5.
Ramanathan RS, Nasr-El-Din HA, Zakaria AS. New insights into the dissolution of iron sulfide using chelating agents. SPE Journal. 2020; 25 (6):3145-3159. Preprint (Preprint): SPE-202469-PA. DOI: 10.2118/202469-PA - 6.
Al-Ibrahim H, AlMubarak T, Almubarak M, et al. Chelating agent for uniform filter cake removal in horizontal and multilateral Wells: Laboratory analysis and formation damage diagnosis. In: Presented at the SPE Saudi Arabia Section Annual Technical Symposium and Exhibition, Al-Khobar, Saudi Arabia, April 21–23, 2015. Texas, USA: OnePetro; 2015. DOI: 10.2118/177982-MS - 7.
Fontana MG, Greene ND. Corrosion Engineering. 1st ed. New York: McGraw Hill Education; 1978 - 8.
Jones DA. Principles and Prevention of Corrosion. 2nd ed. New Jersey: Prentice Hall; 1996 - 9.
Tourky AR, Azim AA, Anwar MM. Effect of carbon content on the corrosion and passivity of iron. Corrosion Science. 1965; 5 (4):301-317. DOI: 10.1016/S0010-938X(65)90636-0 - 10.
Ueda M, Amaya H, Ogawa K, et al. Corrosion resistance of weldable super 13Cr stainless steel in H2S containing CO2 environments. In: Presented at the CORROSION 96, Denver, Colorado, 24–29 March. Texas, USA: National Association for Corrosion Engineers (NACE) International; 1996 - 11.
Cunat P. Alloying elements in stainless steel and other chromium-containing alloys. Euro Inox and International Chromium Development Association. 2004; 1 :1-24 - 12.
Kodama T, Ambrose JR. Effect of molybdate ion on the repassivation kinetics of iron in solutions containing chloride ions. Corrosion. 1976; 33 (5):155-161. DOI: 10.5006/0010-9312-33.5.155 - 13.
Craciunescu C, Hamdy AS. The effect of copper alloying element on the corrosion characteristics of Ti-Ni and ternary Ni-Ti-Cu meltspun shape memory alloy ribbons in 0.9% NaCl solution. International Journal of Electochemical Science. 2013; 8 (2013):10320-10334 - 14.
Townsend HE. Effects of alloying elements on the corrosion of steel in industrial atmospheres. Corrosion Science. 2001; 57 (6):497-501. DOI: 10.5006/1.3290374 - 15.
Amani M, Hjeij D. A comprehensive review of Corrosion and its inhibition in the oil and gas industry. In: Presented at the SPE Kuwait Oil and Gas Show and Conference, Mishref, Kuwait, October 11–14, 2015. Texas, USA: OnePetro; 2015. DOI: 10.2118/175337-MS - 16.
Perez T. Corrosion in the oil and gas industry: An increasing challenge for materials. JOM. 2013; 65 (8):1033-1042. DOI: 10.1007/s11837-013-0675-3 - 17.
Sun W, Nešić S. A mechanistic model of uniform hydrogen sulfide/carbon dioxide corrosion of mild steel. Corrosion. 2009; 65 (5):291-307. DOI: 10.5006/1.3319134 - 18.
Zheng Y, Ning J, Brown B, et al. Mechanistic study of the effect of iron sulfide layers on hydrogen sulfide corrosion of carbon steel. In: Presented at the CORROSION 2015, Dallas, Texas, March 15–19. Texas, USA: National Association for Corrosion Engineers (NACE) International; 2015 - 19.
Abdalsamed I, Amar I, Al-abbasi A, Ahmied E, Farouj A, Kawan J, et al. Hydrogen sulphide strategy in oil and gas field: Review. Scientific Journal for Faculty of Science-Sirte University. 2023; 3 (1):158-165. DOI: 10.37375/sjfssu.v3i1.74 - 20.
De Waard C, Milliams D. Carbonic acid corrosion of steel. Corrosion. 1975; 31 (5):177-181. DOI: 10.5006/0010-9312-31.5.177 - 21.
Nešić S, Sun W. Corrosion in acid gas solutions. In: Richardson JA et al., editors. Sheir’s Corrosion. 2nd ed. Amsterdam, The Netherlands: Elsevier Science; 2010. pp. 1270-1298 - 22.
Nor AM, Suhor MF, Abas AZ, et al. Effect of CO2/H2S on corrosion behavior of API 5L 65 carbon steel in high pCO2 environments. In: Presented at the Offshore Technology Conference-Asia, Kuala Lumpur, Malaysia, March 25–28, 2014. Texas, USA: OnePetro; 2014. DOI: 10.4043/24962-MS - 23.
Fang H, Brown B, Nešic S. High salt concentration effects on CO2 corrosion and H2S corrosion. In: Presented at the CORROSION 2010, San Antonio, Texas, March 14–18. Texas, USA: National Association for Corrosion Engineers (NACE) International; 2010 - 24.
Chang FF, Nasr-El-Din HA, Lindvig T. Matrix acidizing of carbonate reservoirs using organic acids and mixture of HCl and organic acids. In: Presented at the SPE Annual Technical Conference and Exhibition, Denver, Colorado, USA, 21–24 September. SPE-116601-MS; Texas, USA: OnePetro; 2008. DOI: 10.2118/116601-MS - 25.
Finsgar M. Application of corrosion inhibitors for steels in acidic media for the oil and gas industry: A review. Corrosion Science. 2014; 86 (2014):17-41. DOI: 10.1016/j.corsci.2014.04.044 - 26.
Humpston G, Jacobson DM. Principles of Soldering. 1st ed. Ohio: ASM International; 2004 - 27.
Ferhat M, Benchettra S, Amara S, et al. Corrosion behaviour of Fe-C alloys in a sulfuric medium. Journal of Materials and Environmental Science. 2014; 5 (4):1059-1068 - 28.
Vaughn GA, Greer JB. High strength nickel-alloy tubulars for deep, sour gas well application. In: Presented at the SPE Annual Technical Conference and Exhibition, Dallas, Texas, 21–24 September. SPE-9240-MS; Texas, USA: OnePetro; 1980. DOI: 10.2118/9240-MS - 29.
Buijse M, Boer PD, Breukel B, et al. Organic acids in carbonate acidizing. SPE Production & Operations. 2004; 19 (3):128-134. SPE-82211-PA. DOI: 10.2118/82211-PA - 30.
Crowe CW, McGowan GR, Baranet SE. Investigation of retarded acids provides better understanding of their effectiveness and potential benefits. SPE Production Engineering. 1988; 5 (2):166-170. SPE-18222-PA. DOI: 10.2118/18222-PA - 31.
Almubarak T, AlKhaldi M, Ng JH, et al. Matrix acidizing: A laboratory and field investigation of sludge formation and removal of oil-based drilling mud filter cake. SPE Drilling & Completion. 2021; 36 (2):281-299. DOI: 10.2118/178034-PA - 32.
Rostami A, Nasr-El-Din HA. Review and evaluation of corrosion inhibitors used in well stimulation. In: Presented at the SPE International Symposium on Oilfield Chemistry, the Woodlands. Texas, 20–22 April. SPE-121726-MS; Texas, USA: OnePetro; 2009. DOI: 10.2118/121726-MS - 33.
Foster GL, Oakes BD, Kucera CH. Acetylenic corrosion inhibitors. Industrial and Engineering Chemistry. 1959; 51 (7):825-828. DOI: 10.1021/ie50595a027 - 34.
Bockris J. The mechanism of corrosion inhibition of iron in acid solution by acetylenic alcohols. Journal of The Electrochemical Society. 1991; 138 (8):2237. DOI: 10.1149/1.2085956 - 35.
El Dahan H, Mohamed T, Abo El-Enin S. Efficient quaternary ammonium salt as corrosion inhibitor for steel pickling in sulphuric acid media. Anti-Corrosion Methods and Materials. 1999; 46 (5):358-363. DOI: 10.1108/00035599910295571 - 36.
Durnie W, Auty L, Gough M, et al. Characterization, isolation and performance characteristics of imidazolines. In: Presented at the CORROSION 2002, Denver, Colorado, 7–11 April. NACE-02301. Texas, USA: National Association for Corrosion Engineers (NACE) International; 2002 - 37.
Hegazy M, Abdallah M, Awad M, et al. Three novel di-quaternary ammonium salts as corrosion inhibitors for API X65 steel pipeline in acidic solution. Part I: Experimental results. Corrosion Science. 2014; 81 :54-64. DOI: 10.1016/j.corsci.2013.12.010 - 38.
Frenier WW, Hill DG, Jasinski RJ. Corrosion inhibitors for acid jobs. Oilfield Review. 1989; 1 (2):15-21 - 39.
Seth K, Evans BA, Gabrysch AD. Development and testing of a novel corrosion inhibitor technology for acid corrosion. In: Presented at the SPE Middle East Oil and Gas Show and Conference, Manama, Bahrain, 25–28 September. SPE-142675-MS; Texas, USA: OnePetro; 2011. DOI: 10.2118/142675-MS - 40.
Solomon M, Uzoma I, Olugbuyiro J, et al. A censorious appraisal of the oil well acidizing corrosion inhibitors. Journal of Petroleum Science and Engineering. 2022; 215 :110711. DOI: 10.1016/j.petrol.2022.110711 - 41.
Khadom A, Abd A, Ahmed N. Potassium iodide as a corrosion inhibitor of mild steel in hydrochloric acid: Kinetics and mathematical studies. Journal of Bio- and Tribo-Corrosion. 2018; 4 (2):17. DOI: 10.1007/s40735-018-0133-4 - 42.
Al-Katheeri M, Nasr-El-Din H, Taylor K, et al. Determination and fate of formic acid in high temperature acid stimulation fluids. In: Presented at the International Symposium and Exhibition on Formation Damage Control, Lafayette, Louisiana, 20–21 February. SPE-73749-MS; Texas, USA: OnePetro; 2002. DOI: 10.2118/73749-MS - 43.
Cassidy JM, McNeil RI, Kiser C. Understanding formic acid decomposition as a corrosion inhibitor intensifier in strong acid environments. In: International Symposium on Oilfield Chemistry, 28 February-2 March, Houston, Texas, U.S.a. SPE-106185-MS; Texas, USA: OnePetro; 2007. DOI: 10.2118/106185-MS - 44.
Cabello G, Funkhouser G, Cassidy J, et al. Inhibition by CO of the corrosion of Fe, Ni, and their alloys in concentrated HCl solutions. Journal of Electroanalytical Chemistry. 2011; 662 (1):150-156. DOI: 10.1016/j.jelechem.2011.05.015 - 45.
Salimon J, Hernández-Romero R, Kalaji M. The dynamics of the conversion of linear to bridge bonded CO on Cu. Journal of Electroanalytical Chemistry. 2002; 538-539 :99-108. DOI: 10.1016/s0022-0728(02)01052-5 - 46.
Kehrer VJ, Leidheiser H. The catalytic decomposition of carbon monoxide on large metallic single crystals. Journal of Physical Chemistry. 1954; 58 (7):550-555. DOI: 10.1021/j150517a010 - 47.
Broden G, Rhodin TN, Brucker C. Synchrotron radiation study of chemisorptive bonding of CO on transition metals — Polarization effect on Ir(100). Surface Chemistry. 1976; 59 (2):593-611. DOI: 10.1016/0039-6028(76)90038-8 - 48.
Cassidy JM, Wadekar S, Pandya NK. A unique emulsified acid system with three intensifiers for stimulation of very high temperature carbonate reservoirs. In: Presented at the SPE Kuwait International Petroleum Conference and Exhibition, Kuwait City, Kuwait, 10–12 December. SPE-163308-MS; Texas, USA: OnePetro; 2012. DOI: 10.2118/163308-MS - 49.
Hill DG, Jones A. An engineered approach to corrosion control during matrix acidizing of HTHP sour carbonate reservoir. In: Presented at the CORROSION 2003, San Diego, California, 16–20 March. NACE-03121. Texas, USA: National Association for Corrosion Engineers (NACE) International; 2003 - 50.
Sabhapondit A, Vielma JR, Prakash C. Laboratory optimization of an emulsified acid blend for stimulation of high-temperature carbonate reservoirs. In: Presented at the North Africa Technical Conference and Exhibition, Cairo, Egypt, 20–22 February. SPE-150337-MS; Texas, USA: OnePetro; 2012. DOI: 10.2118/150337-MS - 51.
Syafii I, Pandya N, Sabhapondit A, et al. High-temperature acidizing: Advantages of inhibitor-intensifier synergy. In: Presented at the Abu Dhabi International Petroleum Exhibition & Conference, Abu Dhabi, UAE, 7–10 November. SPE-183034-MS; Texas, USA: OnePetro; 2016. DOI: 10.2118/183034-MS - 52.
Al-Mutairi SH, Nasr-El-Din HA, Al-Muntasheri GA, et al. Corrosion control during acid fracturing of deep gas wells: Lab studies and field cases. In: Presented at the SPE International Symposium on Oilfield Corrosion, Aberdeen, United Kingdom, May 13, 2005. Texas, USA: OnePetro; 2005. DOI: 10.2118/94639-MS - 53.
Kalfayan L. Production Enhancement with Acid Stimulation. Tulsa: PennWell Corporation; 2008. p. 5 - 54.
Gaverick L. Corrosion in the Petrochemical Industry. 1st ed. Ohio: ASM International; 1994 - 55.
Smith C, Dollarhide F, Byth NJ. Acid corrosion inhibitors - are we getting what we need? Journal of Petroleum Technology. 1978; 30 (05):737-746. SPE-5644-PA. DOI: 10.2118/5644-PA - 56.
Afra S, Samouei H, Nasr-El-Din HA. NMR investigation of viscoelastic surfactant compatibility with corrosion inhibitors. SPE Journal. 2021; 26 (3):1250-1260. DOI: 10.2118/204214-PA - 57.
Graham GM, Frigo DM, McCracken IR, et al. The influence of corrosion inhibitor/scale inhibitor interference on the selection of chemical treatments under harsh (HP/HT/HS) reservoir conditions. In: Presented at the International Symposium on Oilfield Scale, Aberdeen, United Kingdom, January 30–31, 2001. Texas, USA: OnePetro; 2001. DOI: 10.2118/68330-MS - 58.
Jackson J, Harrington R, Manko D. Emulsion tendency studies -- understanding method, inhibitors and water cut. In: Presented at the CORROSION 2012, Salt Lake City, Utah, March 11–15, 2012. Texas, USA: National Association for Corrosion Engineers (NACE) International; 2012 - 59.
Al-Nakhli AR, Nasr-El-Din HA, Al-Baiyat AA. Interactions of iron and viscoelastic surfactants during well stimulation: A new formation damage mechanism. In: Presented at the SPE Saudi Arabia Section Young Professionals Technical Symposium, Dharan, Saudi Arabia, March 29–30, 2008. Texas, USA: OnePetro; 2008. DOI: 10.2118/117060-MS - 60.
Alotaibi F, Rafie M, Almubarak T, et al. H2S scavengers and corrosion inhibitor interactions for sour crude applications. In: Presented at the International Petroleum Technology Conference, Dhahran, Saudi Arabia, February 12, 2024. Texas, USA: OnePetro; 2024. DOI: 10.2523/IPTC-24603-MS - 61.
Raja P, Sethuraman M. Natural products as corrosion inhibitor for metals in corrosive media — A review. Materials Letters. 2008; 62 (1):113-116. DOI: 10.1016/j.matlet.2007.04.079 - 62.
da Rocha J, da Cunha Ponciano Gomes J, D’Elia E. Corrosion inhibition of carbon steel in hydrochloric acid solution by fruit peel aqueous extracts. Corrosion Science. 2010; 52 (7):2341-2348. DOI: 10.1016/j.corsci.2010.03.033 - 63.
Fidrusli A, Mahmood M. Ginger extract as green corrosion inhibitor of mild steel in hydrochloric acid solution. IOP Conference Series: Materials Science and Engineering. 2018; 290 :012087. DOI: 10.1088/1757-899x/290/1/012087 - 64.
Parthipan P, Elumalai P, Narenkumar J, et al. Allium sativum (garlic extract) as a green corrosion inhibitor with biocidal properties for the control of MIC in carbon steel and stainless steel in oilfield environments. International Biodeterioration & Biodegradation. 2018; 132 :66-73. DOI: 10.1016/j.ibiod.2018.05.005 - 65.
Ng JHC, Almubarak T, Nasr-El-Din HA. Environmentally friendly corrosion inhibitors from leaf extracts. In: Presented at the SPE/AAPG/SEG Asia Pacific Unconventional Resources Technology Conference, Virtual, November 16–18, 2021. Texas, USA: OnePetro; 2021. DOI: 10.15530/AP-URTEC-2021-208324 - 66.
Ng J, Almubarak T, Nasr-El-Din H. Natural alkaloid as a non-toxic, environmentally friendly corrosion inhibitor. The Canadian Journal of Chemical Engineering. 2022; 100 (6):1214-1225. DOI: 10.1002/cjce.24194 - 67.
Almubarak T, Ng JH, Almubarak M, et al. Fragrant flower extracts as corrosion inhibitors in the oil and gas industry. In: Presented at the International Petroleum Technology Conference, Bangkok, Thailand, March 1–3, 2023. Texas, USA: OnePetro; 2023. DOI: 10.2523/IPTC-22877-MS - 68.
Almubarak T, Almubarak M, Rafie M, et al. Turning the most abundant form of trash worldwide into effective corrosion inhibitors for applications in the oil and gas industry. In: Presented at the ADIPEC, Abu Dhabi, UAE, October 31–November 3, 2022. Texas, USA: OnePetro; 2022. DOI: 10.2118/211161-MS - 69.
Almubarak T, AlOtaibi F, Rafie M, et al. Expired medications as corrosion inhibitors in the oil and gas industry. In: Presented at the ADIPEC, Abu Dhabi, UAE, October 2–5, 2023. Texas, USA: OnePetro; 2023. DOI: 10.2118/216036-MS - 70.
Abdallah M, Soliman K, Alfakeer M, et al. Expired antifungal drugs as effective corrosion inhibitors for carbon steel in 1 M HCl solution: Practical and theoretical approaches. ACS Omega. 2023; 8 (38):34516-34533. DOI: 10.1021/acsomega.3c03257 - 71.
Vaszilcsin N, Kellenberger A, Dan M, et al. Efficiency of expired drugs used as corrosion inhibitors: A review. Materials. 2023; 16 (16):5555. DOI: 10.3390/ma16165555 - 72.
Alamry K, Khan A, Aslam J, et al. Corrosion inhibition of mild steel in hydrochloric acid solution by the expired ampicillin drug. Scientific Reports. 2023; 13 (1):6724. DOI: 10.1038/s41598-023-33519-y