Basic properties of various membrane modules.
Abstract
Membrane technology is at the forefront of addressing critical challenges in water scarcity and environmental conservation through advanced wastewater treatment. This paper reviews membrane fouling mitigation strategies in wastewater treatment, highlighting recent advances and future directions. The review underscores the significance of membrane-based processes due to their high efficiency, adaptability, and sustainability. It discusses various membrane classifications, operational modes, and configurations, particularly focusing on cutting-edge developments in membrane-based technologies in wastewater treatment. Membrane fouling, characterized by the undesirable accumulation of particles, microorganisms, and organic compounds on membrane surfaces, impedes membrane performance, leading to permeability loss and increased energy demand. To counteract this, fouling mitigation strategies such as utilizing anti-fouling membrane materials have been at the forefront of research. These strategies aim to maintain membrane efficacy and longevity, thereby ensuring the sustainability of membrane-based wastewater treatment systems. Future research anticipates a paradigm shift toward smart membrane systems, utilizing external potentials and advanced material properties to address fouling and optimize performance, representing the next frontier in sustainable wastewater management.
Keywords
- membrane
- wastewater treatment
- fouling
- fouling mitigation
- membrane surface modifications
- nanoparticle coatings
1. Introduction
Water scarcity is a pressing global issue, profoundly impacting both human health and environmental sustainability. With the ever-increasing human population and its correlated demands on water resources, traditional water sources are being depleted at an alarming rate. This depletion is further exacerbated by factors such as economic development, population explosion, climate change, and expansion of irrigated agriculture [1]. Concurrently, the generation of wastewater from domestic, industrial, and agricultural activities has escalated, creating a critical need for effective wastewater treatment methods [2]. Membrane technology, as a versatile and efficient separation process, has emerged as a key solution in addressing these challenges. Membrane technology is not only integral in the treatment of wastewater but also plays a crucial role in the purification and formulation of products across a wide array of sectors including water, energy, food, and environmental industries [3].
1.1 Fundamentals of membrane technology
The fundamentals of membrane technology in wastewater treatment revolve around membrane classification, materials and fabrication techniques, operation principles and systems [4]. A membrane is a molecular sieve built into the structure of a film with/without small pores or fine mesh to facilitate the separation of small particles and molecules [5]. Membranes are typically classified based on their pore size, driving force, morphology, and material composition, which can be either organic or inorganic. The pore size is a critical determinant in membrane selection, influencing its suitability for separating different components in a mixture [6]. The operational advantage of membrane technology in wastewater treatment is primarily its high efficiency in treating feed mixtures that are difficult to handle with conventional separation methods (e.g., adsorption or settling). This efficiency is due to the selective nature of the membrane, allowing for the differentiation of components based on size or affinity [7]. Its principle lies in the use of a semi-permeable barrier, which separates undesired components from a feed solution under various potential gradients, for example, pressure, temperature, electrical, or concentration differences. This technology stands out for its ability to produce stable products without the addition of chemicals and its relatively low energy consumption, making it a sustainable option for wastewater treatment [8]. Additionally, its modularity and scalability allow for flexible application across various treatment capacities, while its compact and simple design eases implementation and maintenance [9].
1.2 Classification of membranes
In the field of wastewater treatment, membrane-based technologies have become central solutions owing to their effectiveness and versatility in addressing diverse treatment requirements. These technologies include a range of membrane processes, specifically microfiltration (MF), ultrafiltration (UF), nanofiltration (NF), and reverse osmosis (RO), each distinguished by unique functionalities and applications [10]. Figure 1 presents the range of membrane processes including the average diameter of the pores and the relative size of different solutes removed by each membrane class. Microfiltration and ultrafiltration are used in the separation of emulsions, silts, and oils from wastewater [11]. Their efficacy in these applications is enhanced when combined with other technologies, broadening their implementation scope. For instance, modified ultrafiltration membranes, developed from materials like polyvinylidene fluoride (PVDF) and titania nanotubes (TNT), have demonstrated significant color removal efficiencies in industrial wastewater treatments, such as those from palm oil mills [8]. Nanofiltration plays a vital role in rejecting divalent ions while allowing the passage of monovalent ions like Na+ and Cl− [12]. This makes NF particularly effective in recovering heavy metals from water, as most heavy metals are bivalent or higher [10]. NF effectively softens water by selectively removing hardness-causing ions, such as calcium and magnesium, while retaining beneficial minerals and consuming less energy than traditional methods. The technique’s ease of operation, low energy consumption, high efficiency, and reliability make it a promising option for treating various types of wastewater. Reverse osmosis uses dense (non-porous) membranes for water separation and is widely implemented for seawater desalination and for the management of micropollutants such as perfluoroalkyl substances (PFASs) in aqueous solutions, which are detected in various industrial products and have been associated with health and environmental risks [3]. In addition to these specific applications, the integration of different membrane technologies is often preferable to overcome the limitations of each process, conserve energy, and increase overall performance efficiency [9]. The cooupling of MF or UF with NF, and RO technologies, for instance, was shown to enhances treatment of oil-produced water, meeting environmental legislative requirements [4].
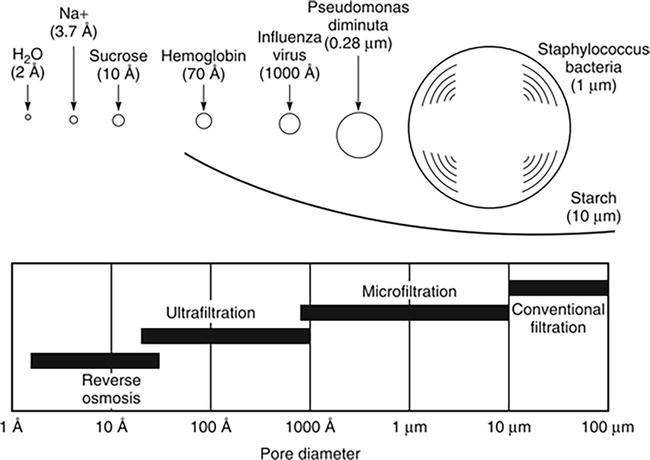
Figure 1.
Traditional filtration, microfiltration, ultrafiltration, and reverse osmosis represent a spectrum of related membrane-based processes, primarily distinguished by the average diameter of the pores in their respective membrane filters. This schematic illustrates the relative size of different solutes removed by each membrane class. Modified from baker [
1.3 Operational modes and membrane modules
Membrane technology primarily operates by two distinct modes: dead-end and cross-flow filtration. Dead-end filtration (Figure 2) involves the direct flow of the feedwater perpendicular to the membrane surface. As elucidated in the studies by Wang et al. [13], this mode can be employed in gravity-driven membrane (GDM) systems for drinking water treatment. The primary characteristic of dead-end filtration is the accumulation of a contaminant layer or ‘cake’ on the membrane’s surface, which can lead to increased resistance and reduced flow over time. This mode is generally more straightforward and less energy-intensive but requires regular maintenance to manage the cake buildup and prevent membrane fouling, resulting in a ‘batch mode’ operation pattern. Cross-flow filtration (Figure 2) introduces the feedwater parallel to the membrane surface, creating a shear that minimizes the accumulation of contaminants. The cross-flow mechanism allows for a continuous operation with a more stable flux, as the tangential flow constantly removes the particles from the membrane surface. However, this mode typically demands higher energy inputs due to the need for continuous flow across the membrane. This mode, as highlighted by Díaz et al. [14], is particularly effective in applications like anaerobic membrane bioreactors (AnMBRs), where the prevention of membrane fouling is crucial for maintaining flux. The choice between dead-end and cross-flow filtration is often dictated by the specific requirements of the process, including the nature of the feedwater, desired purity levels, and operational costs [15].
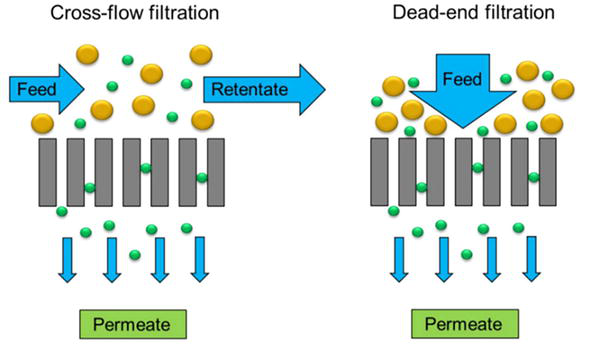
Figure 2.
Configurations of cross-flow filtration (left) and dead-end filtration (right). Adapted from Wang et al. [
The selection of membrane modules (Table 1) is pivotal, governed by their design and operational characteristics. Spiral Wound (SW), Hollow Fiber (HF), Tubular, and Flat-sheet modules offer unique advantages [12]. SW modules are industry standards in RO/NF, HF is predominant in wastewater treatment, Tubular modules excel in high-solids wastewater treatment, and Flat-sheet designs are favored for small to medium-scale applications with turbid wastewater [8]. Furthermore, the choice of membrane module is informed by quality requirements, fouling tendency, and operational lifespan [15]. Quality requirements hinge on the specific water treatment needs, while the fouling tendency, a critical consideration, varies with feedwater type and includes strategies like hydrodynamic operation and chemical cleaning for prevention [3]. Operational lifespan, an economic factor, varies across membrane types, influencing the overall efficiency and cost-effectiveness of water treatment systems [8].
Property | Plate-and-Frame | Tubular | Spiral Wound | Hollow Fiber | Ref. |
---|---|---|---|---|---|
Packing Density ft2/ft3 (m2/m3) | 45–150 | 6–120 | 150–380 | 150–1500 | [8] |
(148–492) | (20–374) | (492–1247) | (492–4924) | ||
Potential for fouling | Moderate | Low | High | Very High | [12] |
Ease of Cleaning | Good | Excellent | Poor | Poor | [3] |
Relative Manufacturing cost | High | High | Moderate | Low | [15] |
Table 1.
2. Membrane-based technologies for wastewater treatment
Advancements in wastewater treatment and reuse technologies are essential for achieving optimal water quality, a critical factor in various applications. Accordingly, a vast spectrum of treatment processes are tailored for water reuse applications, each designed to enhance effluent quality to meet diverse requirements for water reuse scenarios [3]. Traditional wastewater treatment methodologies typically rely on biological processes to eliminate biochemical oxygen demand (BOD), total suspended solids (TSS), and nutrients such as nitrogen in terms of ammonia and nitrate, and phosphorus. However, in membrane-based technologies used for wastewater treatment, implementing mainly UF and MF membranes, the role of membranes becomes increasingly pivotal, not only in removing residual suspended solids for tertiary treatment but also in augmenting the efficiency of disinfection methods as UF membranes are selective to bacteria, parasites, and some viruses [10]. Their adaptability, coupled with environmental sustainability and cost-effectiveness, positions membrane-based processes at the forefront of advanced wastewater treatment solutions, aligning with the growing demand for high-quality, reusable water (by tertiary treatment). The next chapter reviews technologies coupling membranes with the biological, electrical and thermal processes to improve wastewater treatment.
2.1 Membrane bioreactors
Membrane Bioreactors (MBRs) have revolutionized wastewater treatment by integrating biodegradation and advanced filtration technologies. At their core, MBRs combine activated sludge treatment MF or UF, using porous membranes to achieve superior separation of liquid and solid phases [16]. This results in several significant enhancements compared to conventional activated sludge processes [6], including reducing the overall footprint of the treatment system due to compact membrane modules, enhancing the quality of effluent, and providing thorough retention of bacterial flocs and suspended solids, thereby ensuring effective disinfection [8] and improving the system’s ability to operate under high mixed liquor-suspended solids concentrations and extended solid retention times (SRT), thus enabling improved wastewater quality and the treatment of specific organic pollutants, particularly those nitrogen-based, while reducing the bioreactor size [4].
The development of MBR systems has seen significant technological advancements including the introduction of submerged membrane configuration, which involved placing the membrane directly within the bioreactor and employing aeration to minimize fouling, significantly lowering operational expenses and streamlining the treatment process, thereby catalyzing the widespread acceptance of MBR technology (Figure 3) [8]. Submerged MBRs come in various configurations, including vertical flat plates and hollow fibers, each with unique advantages and considerations regarding fouling and cleaning [17]. The operational aspects of MBRs, such as solid and hydraulic retention times, are critical and influence the nature of the activated sludge [16]. These systems can operate at lower HRT, resulting from decoupling them from SRT [18]. The intensive biological degradation capability of MBRs, coupled with their ability to retain biomass, facilitates efficient pollutant removal and, in some cases, complete nitrification. Furthermore, MBRs can effectively remove nitrogen and phosphorus through denitrification and chemical or biological processes, respectively [4]. As a result, MBRs have found applications across various industries including for treating high-strength wastewater, that pose challenges to conventional treatment processes [18]. Additionally, MBR can be paired with advanced treatment methods, e.g., activated carbon, post-ozonation, or reverse osmosis, for further purification and reuse, including groundwater recharge and surface water augmentation [6].
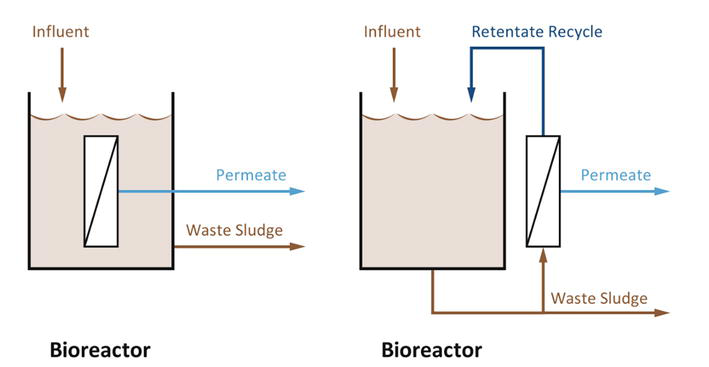
Figure 3.
Schematic configuration of side-stream (non-submerged) and submerged MBRs.
While aerobic MBRs are typically considered for wastewater treatment, anaerobic membrane bioreactors (AnMBR) are a good alternative due to their low energy demand. AnMBRs combine anaerobic biological treatment with membrane filtration [19] with varying configurations (Figure 4). AnMBR processes leverage the inherent advantages of anaerobic treatment, including low energy requirements and biogas production, with the added benefit of membrane-based solid-liquid separation [21]. This results in a system with a smaller footprint, higher organic removal rates, and improved effluent quality compared to conventional treatment methods [20]. AnMBRs are particularly adept at handling municipal wastewater, industrial effluents, and high-strength organic wastes, making them versatile for various applications [22]. Recent advancements in AnMBR include integration with forward osmosis (FO) systems for pretreatment, which shows promise in reducing biofouling and enhancing membrane longevity [16]. AnMBRs also employ various membrane materials and configurations, such as hollow fiber, flat sheet, and multi-tubular membranes, each with distinct performance characteristics and operational parameters [8]. The integration of AnMBR with other treatment systems, such as microalgae cultivation and microbial fuel cells, offers promising avenues for nutrient recovery and energy efficiency improvements [19]. One of the key challenges in AnMBR technology is membrane fouling, which can hamper operational efficiency and productivity [20]. Fouling is typically caused by the accumulation of organic and inorganic matter and biological colloidal particles on the membrane surface. As AnMBRs have limited/no air scouring, they are more prone to fouling accumulation. Various strategies have been developed to mitigate fouling, such as increasing HRT and SRT and employing physical and chemical cleaning methods [21].
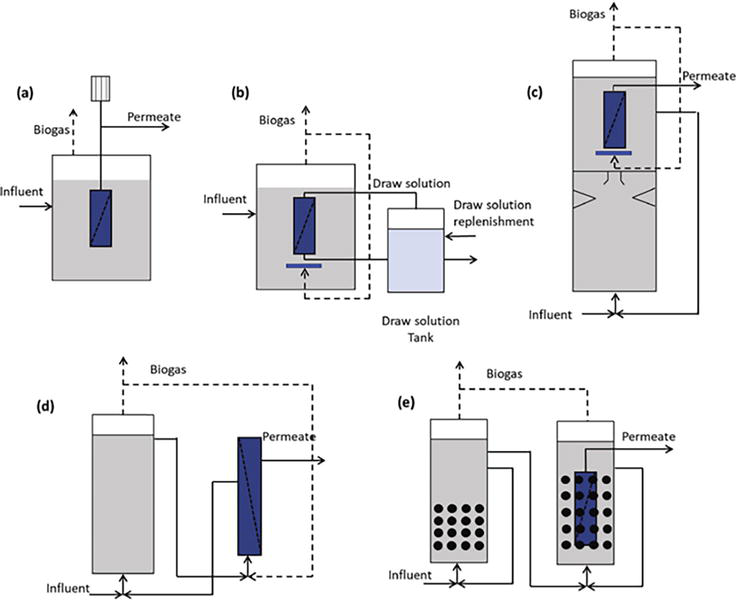
Figure 4.
AnMBR configurations for wastewater treatment. (a) Rotating membranes; (b) anaerobic osmotic membrane bioreactor; (c) membrane coupled at the top of the AnMBR-UASB; (d) gas-lift AnMBR; (e) two-stage anaerobic fluidized membrane bed bioreactor. Adapted from Vinardell et al. [
Apart from the biological treatment of wastewater by MBRs, we present several membrane technologies based on driving forces related to thermal and electrical gradients which are not very common but may have applications in wastewater treatment.
2.2 Membrane distillation
Membrane Distillation (MD) is a thermal membrane process that involves the separation of substances based on their partial vapor pressure [4]. Water vapor is transported across a hydrophobic microporous membrane for wastewater treatment, driven by a vapor pressure gradient [8]. MD is especially effective for feed solutions with higher salinity and utilizes a low-grade thermal energy based gradient (around 50–70°C) to create the necessary vapor pressure difference across the membrane [23]. The technology has several advantages, including the ability to use solar heating and waste heat from industrial processes to drive the separation process [15]. MD operates under very low hydrostatic pressure compared to RO or even MF, leading to reduced membrane fouling as only water vapor is transported across the membrane, and therefore, bacteria are not driven to the membrane by permeated drag force [6]. Furthermore, due to the elevated temperatures, some bacterial strains do not survive, and only thermophilic bacteria will eventually be able to form biofilms [24]. MD can efficiently separate nonvolatile materials from volatile ones, with feed product separation reaching 100% [3]. However, MD faces challenges as membrane wetting, resulting from fouling (scaling and biofouling), leading to rejection decline and possible damage to the membrane [25]. Avoiding such processes requires extensive feed water pretreatment, which increases operational costs [8]. Moreover, simultaneous heat and mass transfer in MD can cause temperature polarization, negatively impacting the driving force and reducing permeate flux [4]. MD has been applied in various contexts, such as treating water from the Arabian Gulf and table olive wastewater, showing promise in achieving high permeate flux and energy utilization [23].
Incorporating MD into wastewater treatment from aquaponics and hydroponics systems offers significant potential for enhancing water management and sustainability [26]. For example, for coupling aquaponic systems, MD treated water from the hydroponic units can be purified and reused in recirculating aquaculture systems (RAS) [27]. Moreover, MD’s relatively low thermal energy requirement (in comparison with distillation) aligns well with these systems’ energy profiles, where waste heat from other processes can be harnessed [4].
2.3 Electrodialysis
Electrodialysis (ED) is a membrane separation process that relies on the movement of ions across selective membranes under the influence of an electrical field [28]. It consists of an array of alternating cation and anion exchange membranes (Figure 5). When a feed solution containing various ions passes through the system, cations move toward the cathode and anions toward the anode, traversing their respective exchange membranes [29]. This process effectively separates ions from the feed solution, concentrating them in different compartments. ED’s application in wastewater treatment is multifaceted [8]. For example, it can selectively remove phosphates from wastewater by using a combination of selective and nonselective membranes [11], demonstrating the versatility of ED in targeting specific ions for the removal and recovery of compounds with economic value. Another example of selective removal is the removal of arsenic from brackish water [28]. The selectivity in removing monovalent anions over di- and multivalent anions is due to steric effects and different charge interactions [11]. This selectivity allows for the optimization of the process for specific applications, such as nutrient removal from wastewater. For instance, a more than 70% removal rate for monovalent ions can be achieved, compared to a removal rate below 50% for divalent ions [29]. This highlights ED’s effectiveness in selective ion removal and its potential for customization in various wastewater treatment scenarios [10]. Additionally, ED can be used to treat concentrated brine from RO in desalination plants or treat saline water, thus reducing brine discharge and using an additional water source [3]. The energy requirements for ED in industrial wastewater applications vary, typically ranging from 6 to 11 kW h/m3 of feed stream, depending on the water reclamation rate and water composition [29].
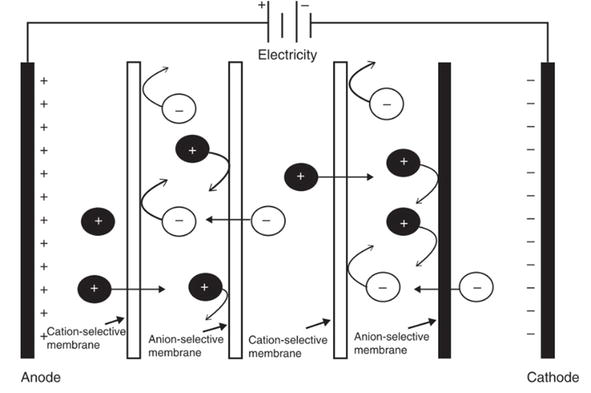
Figure 5.
A basic electrodialysis system for wastewater treatment. (Source: [
2.4 Integration of membranes in aquaculture wastewater treatment
Aquaculture effluents present unique challenges due to high levels of nutrients and suspended solids, necessitating efficient treatment for environmental protection and water reuse [27]. Membrane technology, including MF, UF, and MBRs, plays a crucial role in aquaculture wastewater treatment [10]. These systems efficiently remove fine solids, bacteria, and nutrients, enabling water reuse in aquaculture systems. Studies show that ceramic membranes with Ti–Mn/TiO2/Al2O3 coatings effectively remove suspended solids, achieving up to 100% turbidity removal and reducing Total Ammonia Nitrogen (TAN) to below 0.1 mg/L [30]. MBRs and capillary microfiltration effectively manage organic loads, with a Chemical Oxygen Demand (COD) removal efficiency of 52.1% [13]. The use of superhydrophobic membranes in MD is crucial for treating high-salinity aquaculture wastewater [4] and demonstrates superior wetting resistance, which is essential for long-term operation [3]. However, challenges such as membrane fouling and operational optimization remain significant. For instance, while the integration of MBRs in RAS has notably enhanced water quality and reduced the need for water exchange, it has not substantially improved fish survival or growth, underscoring the necessity for additional research to refine the efficiency and cost-effectiveness of these systems [30, 31].
Another innovative water treatment technology coupling membrane with RAS integrates nutrient assimilation into microbial biomass production (Figure 6), offering environmental benefits over conventional RAS, which relies on nitrification. Using a microaerophilic membrane assimilation reactor (MMAR-RAS), this new approach reduces greenhouse gas emissions and nutrient discharge, lowers energy demands, and utilizes nutrients to produce protein-rich biomass [32]. This biomass is then converted into high-protein fish feed with health-promoting properties [33]. However, the MMAR-RAS faces challenges due to the accumulation of fine suspended solids. The integration of ultrafiltration membrane technology is proposed to address this issue, enhancing the efficiency and sustainability of the system.
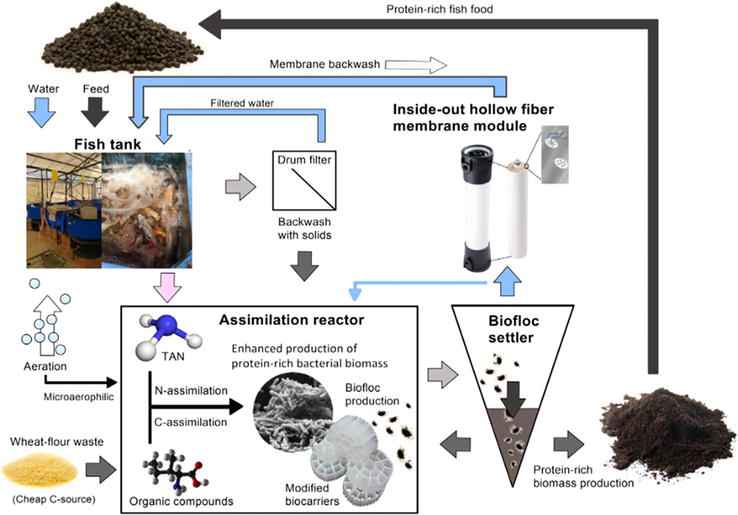
Figure 6.
A novel treatment of intensive fish wastewater by ultrafiltration membrane technology.
3. Membrane fouling
Although membranes have many advantages, as described above, all membrane processes suffer from fouling, which is predominantly caused by the physical and chemical interactions between the membrane surfaces and foulants. Mass transport and operational conditions (including temperature, pH, ionic strength, permeate flux, and cross-flow velocity) may contribute to foulant attachment, accumulation, or adsorption on the surfaces of membranes [34]. Generally, fouling could result from the presence of foulants such as organic matter, colloids, microorganisms, ions, or a mixture of them in the treated wastewater. As the membranes are typically porous, inorganic fouling (scaling) is not considered a significant fouling contributor.
The interactions between foulants and membrane surface can be physical or chemical depending on the properties and other operational parameters, resulting in pore blocking, cake formation, organic adsorption, or biological membrane fouling as shown in Figure 7. Depending on the interaction between the membrane surface and the foulants present in wastewater, membrane fouling can be classified as reversible or irreversible. In the case of reversible fouling, membrane flux can be restored through backwashing or by using a particular chemical cleaning (e.g., chlorine or surfactants) including by cleaning in place (CIP). Nevertheless, the chemisorption or pore plugging due to the presence of foulants on the membrane surface may result in permanent, irreversible membrane fouling. In this case, the reduction in membrane performance (permeate flux loss) cannot be recovered chemically or hydrodynamically [36]. The following subsection provides an overview of different kind of membrane fouling and their impact on membrane performance.
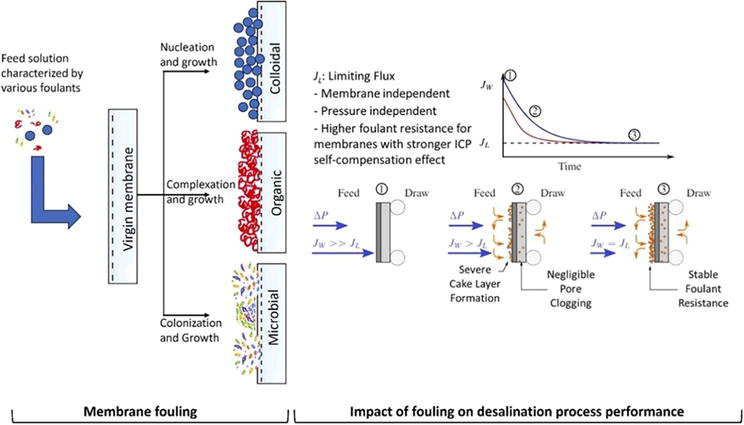
Figure 7.
The process of membrane fouling and its impact on the membrane process performance. (Source: [
3.1 Colloidal and particulate fouling
Colloidal or particluar membrane fouling occurs when the particles present in the feedwater deposit onto the surface of the membrane, forming a fouling cake layer [35]. Cake formation often depends on the nature of the particles present in the wastewater. The presence of the cake layer significantly affects the efficacy of the membrane performance and leads to a decrease in membrane flux or an increase in transmembrane pressure (TMP). The formation of a cake layer may also result in a gel layer and elevated ion concentration near the membrane, leading to a phenomenon described as concentration polarization (CP), which may further lead to inorganic scaling if ions concentration exceeds their solubility limit [36]. Colloids are particles ranging from nanometers to micrometers in size and are one of the common foulants in aquatic environments. They include silt, clay, small organic compounds, and silica [37]. A specific case of particulate fouling occurs by colloids resulting from their small diameter. Collidial transport is governed by convection, Brownian diffusion, shear-induced diffusion, inertial lift, gravitational settling, and lateral migration [38]. Generally, membrane fouling by colloidal particles is attributed to three main factors: pore blockage, cake formation, and concentration polarization [39]. Whenever colloids contact the porous membrane surface, they first deposit onto the surface and block its pores; as additional particles continue to further deposition onto this initial layer, a cake layer forms. This cake layer controls the overall transport and efficiency of membrane performance, resulting in an increment in the TMP and a decrement in permeate flux. Colloidal fouling may also lead to CP, resulting in back diffusion and possible scaling [10, 36, 40].
3.2 Organic fouling
Organic fouling occurs from the presence of organic matter in the treated wastewater, this inlcudes proteins, humic acids, other organic acids and additional organic components such as natural organic matter (NOM). These compounds can accumulate on the membrane’s surface or inside membrane pores, leading to a decrease in flux and possible formation of a “gel layer” when the applied pressure compacts the NOM on the membrane’s surface. The presence of bacteria and biofilms may also lead to further extraction of organic matter and recution in filtration abilities. Overall, organic fouling may result in a gel/cake layer formation, physical obstruction of pores and CP. Organic fouling is also affected by operating variables such as temperature, pressure, and flow rate in addition to membrane characteristics such as surface charge, pore size, and hydrophobicity [24, 41]. As organic coumpound in the feed water vary between sources and the types of wastewater, impacting foulant types and concentrations, it is vital to have an in-depth understanding on the properties of the feed to allow designing efficient strategies (e.g., coagulation and separation, dissolved air floatations) that specifically target the organic compounds in order to prevent membrane fouling.
3.3 Biological fouling
Biofouling is considered the ‘achiless heel’ of membrane filtration and is a significant issue in membrane applications. Biofouling is characterized by the buildup of microorganisms at the interface between phases, for example, the phase between liquid (wastewater) and solids (a polymeric membrane). Bacterial cells deposit, develop, and metabolize on the membranes, leading to surface conditioning, adherence, microcolonies, and maturation of mature biofilms, as shown in Figure 8 [42]. Biofilms consist of multiple layers of microbial cells (dead and active) and extracellular materials secreted by them. Cell biomass and extracellular polymeric substances (EPS) comprise 50–80% of biofilms’ organic matter and proteins [43]. Biofouling can affect membrane performance in all membrane types, leading to a decrease in membrane permeability, increased pressure requirements, and elevated salt passage due to CP. Proteins and carbohydrates are two main macromolecular components contributing to biofilm production. In addition, shorter polymeric structures are secreted by bacteria or break down from the EPS to from transparent exopolymer particles (TEPs). TEPs are small particles and do not tend to agglomerate, so they are challenging to remove using conventional pretreatment procedures. Therefore, small bacterial precursors produce fouling on RO membranes even after removing large particles [44]. The use of biocides was shown only slightly efficiecnt for biofouling mitigation as the EPS provides as a buffer and protective layer for bacteria once it is formed. Additional approaches such as pretreatment of the wastewater aimed at lowering bacteria and nutrient concentrations. Low levels of biofilm formation rate (BFR) and assimilable organic carbon (AOC) have been linked to lower risks of biofouling [45]. Phosphate limiting is an additional technique for managing biofouling of spiral wound RO membranes. Overall, understanding biofilm characteristics and behavior is necessary to design more effective methods for managing membrane biofouling [46]. It should be noted that membrane fouling resulting from organic and biolofical factors is a significant problem for all MBRs as bacterial and organic matter concentrations in the reactors are very high.
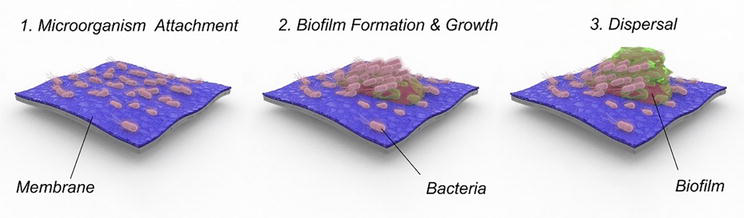
Figure 8.
Biofilm formation steps, (1) attachment to the surface, often preceded by a surface conditioning step and/or by deposition of organic material, (2) growth and creation of microcolonies, and (3) expansion of the biofilm to other areas, often followed by a detachment of part of the biofilm and dispersal. (Source: [
4. Fouling mitigation strategies
As discussed in the previous section, membrane fouling is mainly caused by ions, particles, and bacteria in wastewater systems. However, wastewater typically contains a mixture of these foulants. Consequently, numerous initiatives have been undertaken to tackle the issue of membrane fouling. For example, effective per-treatment of wastewater, timely backwashing of membranes, chemical cleaning, optimization of operating conditions, membrane surface modification, development of advanced membrane materials, and in-line fouling monitoring.
Three promising fouling mitigation approaches, which are membrane surface modification, optimization of operation conditions, and advanced fouling mitigation strategies, are discussed in detail as follows.
4.1 Membrane surface modifications
Membrane surface modification is considered a common strategy to reduce membrane fouling. During membrane modification, controlling surface roughness, membrane surface charge, hydrophilicity, and hydrophobicity play a significant role in reducing possible fouling tendency. Generally, membranes with hydrophilic and smooth surfaces demonstrate less fouling [47]. Among the surface modification possibilities, a few approaches are described in Table 2. The following section discusses three approaches to reduce fouling by surface modification.
Membrane Surface Modifications | Modification method | Membrane details | Modifier | Foulant | Parameters changed | Comments on characteristics | Ref. |
---|---|---|---|---|---|---|---|
Application of anti-fouling coatings | Dip coating | Commercial PA TFC membrane by Woongjin Chemical Co., Ltd. (Korea) | MMA-HPOEM | BSA, | Increased hydrophilicity and the results demonstrated that the coating layer was durable to chemical cleaning. | Coating resistant to chemical cleaning; lower initial flux; enhanced anti-fouling characteristics | [48] |
Layer-by-layer assembly | Commercial RO membrane (ES-20; Nitto Denko, Japan) | Polyelectrolyte | BSA | Enhanced hydrophilicity and surface smoothness | Enhanced hydrophilicity and surface smoothness | [49] | |
Spray coating | Commercial PA RO membrane | TiO2 NPs | Humic acid | Increased membrane hydrophilicity and negative charge | Increased membrane hydrophilicity and negative charge | [50] | |
Commercial PA TFC membrane | P(NIPAM-co-Am) | BSA | Increased membrane surface hydrophilicity | Higher permeability and resistance to BSA fouling; improved cleaning efficiency | [51] | ||
Embedment of polymeric brushes | Interfacial polymerization with TMC | Polysulfone ultrafiltration substrates | Hydrophilic zwitterionic striped structure | Humic acid (HA), BSA and sodium alginate (SA) | Improved hydrophilicity | High selectivity and outstanding anti-fouling ability | [52] |
Grafting onto polydopamine activated membrane | PES Ultrafiltration 20 kDa | Thiolated zwitterionic polyurethane | BSA | Improved hydrophilicity | Exceptional fouling resistance | [53] | |
Layer-by-layer assembly | Commercial NF membrane (NF 90) | Amino-Quinone networks-Phytic acid pseudo Zwitterionic | BSA | Improved hydrophilicity | Excellent fouling resistance and high membrane cleaning efficiency | [54] | |
Surface enhancement using nanoparticles | Vacuum filtration | Polyacrylonitrile (PAN) ultrafiltration substrate | TiO2 | Na2SO4 | Crumpled and thinner PA layer with more uniform structure | An increased permeability by 20.8% than that of TFC membrane and a Na2SO4 rejection of 98.2% | [55] |
Brush painting | Poly(ether sulfone) microfiltration | SWCNT | Na2SO4 | Ultrathin PA layer with higher cross-linking degree, and increased negative surface zeta potential | High water permeability of 40 L·m − 2 ·h − 1 ·bar−1, high Na2SO4 rejection of 96.5% | [56] | |
Spray coating | Ultrafiltration PSF membrane | MXene | Salt rejection | Thinner polyamide layer with increased hydrophilicity and roughness | Nearly double fold of water flux, higher selective separation factor, outstanding salt rejection (>97%) | [57] |
Table 2.
Studies related to membrane surface modification using different modification methodologies.
4.1.1 Application of anti-fouling coatings
Membrane coating using polymers promoting anti-fouling has been explored. The polymers are designed to reduce bacterial and organic matter adhesion by increasing surface hydrophilicity or proving anti-fouling abilities via higher surface negative charge, and surface smoothness.
For example, Zhao et al. used polydopamine (PDA) to modify the membrane’s surfaces and improve its hydrophilicity. However, this method often results in decreased water permeance due to adding a layer with lower permeability on the pristine membrane. Therefore, a pressure-assisted coating method was developed to tune the inner structure of membranes, enhancing water permeance and salt rejection [58]. In another research, Jee et al. modified a spiral-wound RO membrane with a polysiloxane coating with 3-glycidoxypropyltrimethoxysilane (GPPTMS) to increase its hydrophilicity, resulting in improvment in fouling resistance. The study found that fouling decreased with GPPTMS concentrations over 1.0 wt% [59]. Xu et al. showed that loose nanofiltration membranes (LNMs) are promising for dye/salt separation, but membrane fouling hinders real-world applications (Figure 9). Therefore, they prepared a new anti-fouling LNM, by co-deposition of diacrylamide (AMTHBA) and polydopamine (PDA) onto polysulfone (PSf) membrane, thus improving the membrane’s hydrophilicity. The optimum PSf/PDA-AM 1.5 LNM achieved high water permeance, excellent dye rejection, and low salt retention. It also exhibited outstanding anti-fouling properties against organic foulants and bacteria [60]. Qiu et al. reported fabrication of a modified PES membrane where a cationic antibacterial agent, quaternary ammonium compound (QAC), was integrated into a MgAl layered double hydroxide (QAC/LDH) composite, enhancing its hydrophilicity, water flux, and resistance to organic fouling. The PES-QLDH membrane showed superior antibiofouling performance, inhibiting bacteria adhesion and growth. Its synergistic effect resulted in high biocidal activity, making it a promising strategy for wastewater treatment [61].
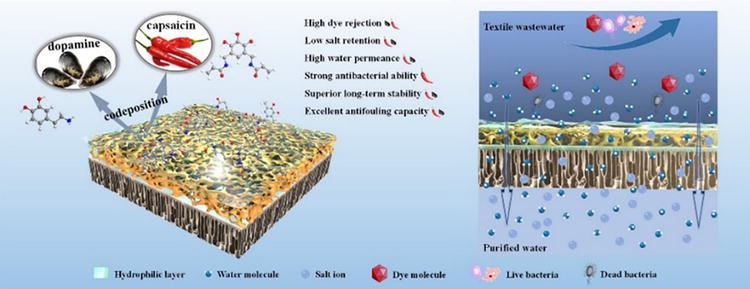
Figure 9.
Representation of membrane modification and its applicability for anti-fouling capability. (Source: [
4.1.2 Embedment of polymeric brushes
Industrial membranes are fabricated from robust, hydrophobic or slightly hydrophobic polymers such as polyethersulfone (PES), polyvinylidene fluoride (PVDF), and polysulfone (PS), which are more prone to fouling. Consequently, hydrophilic polymer brushes can impact fouling potential resulting from improved hydrophilicity and steric interferences [62]. Novel brushes are based on zwitterionic polymers with two opposing charged moieties, yet their hydrophilicity, chemical stability, and “salting-in” action make them useful for anti-fouling applications. They are often used in grafting to create a hydration layer that diminishes fouling and raises the membrane’s hydrophilicity. Water molecules were shown to form bonds with zwitterionic brush chains as they include both positively and negatively charged moieties. Furthermore, these materials promote unhindered mobility and prevent the accumulation of foulants [62]. For example, Song et al. reported the utilization of novel thiolated zwitterionic polyurethane (TZP) and its grafting onto a polydopamine-activated membrane surface for the preparation of engineering anti-fouling nanofiltration (NF) membranes. The chemical structure was confirmed by 1H nuclear magnetic resonance and the grafting improved membrane hydrophilicity. The pore size and ion selectivity of the NF membranes were optimized to achieve divalent anion retention, and excellent fouling resistance (Figure 10a). Figure 10c, d, presents the grafted polymer brushes onto the engineered NF membrane and the repulsion of organic fouling agent (Bovine Serum Albumin) away from the membrane surface. After grafting, the formation of a hydration layer also improved water flux by about 10–20% (Figure 10b) [53].
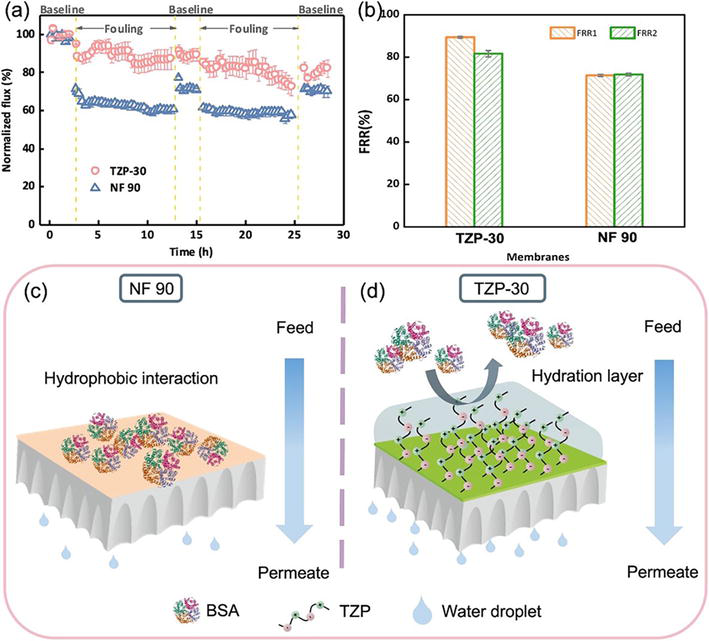
Figure 10.
(a) Normalized water flux of the TZP-30 and NF 90 membranes as a function of cumulative filtration time during two cycles of dynamic fouling tests. Synthetic wastewater, including 0.94 mM NH4Cl, 0.45 mM KH2PO4, 0.5 mM CaCl2, 0.5 mM NaHCO3, 2.0 mM NaCl, 0.6 mM MgSO4, and 100 mg L−1 BSA, was used as feed solution, and the initial flux of membrane was set at 50 L m−2 h−1; (b) flux recovery ratios of the TZP-30 and NF 90 membranes after each fouling cycle; (c–d) schematic illustration of the interaction between foulants and membrane surface: (c) NF 90 membrane and (d) TZP modified membrane. (Source: [
In addition, Deng et al. fabricated an anti-fouling thin-film composite nanofiltration membrane using zwitterionic polyethyleneimine (PEI) moieties. The membrane showed stronger hydration capacity and thicker hydration layer, improved water permeability by 11.6%, and improved ion selectivity. The zwitterionic PEI grafted on the TFC NF membrane mitigated electrostatic attraction and improved anti-fouling properties [63]. Zhang et al. proposed a difunctional nanofiltration membrane with anti-fouling and antibacterial properties (Figure 11) by grafting a random terpolymer with quaternary ammonium and zwitterionic moieties fabricated by free radical polymerization. The modified membrane inhibits protein and bacterial adhesion and shows high bacteria-inhibiting efficiency [64].
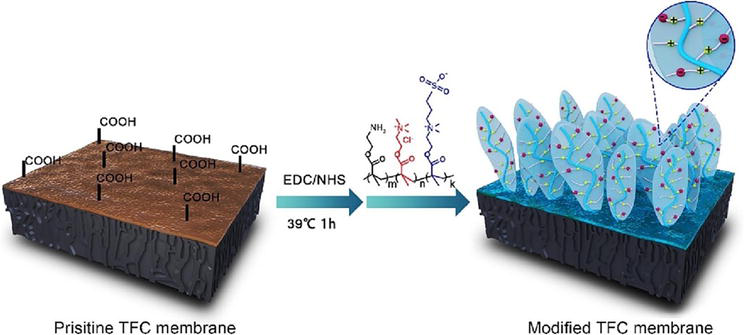
Figure 11.
Schematic illustration of grafting bifunctional terpolymer to the TFC nanofiltration membrane surface. (Source: [
4.1.3 Surface enhancement using nanoparticles
Apart from polymer-based modifications, research efforts have been directed toward creating nano-enhanced membrane surfaces by leveraging the hydrophilic properties of nanomaterials. These include carnon based Np such as Graphene Oxide (GO), Carbon Nanotubes (CNTs), and Metal-Organic Frameworks (MOFs), Metal Nanoparticles (Metal NPs, including metal nanoparticles and metal oxides), and Mxenes. Nanomaterials are recognized for their high aspect ratio and outstanding electrical and thermal conductivities. Researchers worldwide have explored the incorporation of these nanomaterials into membrane filtration processes. Several recent review articles delve into the applications of these nanomaterials in enhancing membrane performance [65, 66, 67, 68, 69, 70].
For example, recently, Firouzjaei et al. [71] prepared a thin-film nanocomposite (TFN) membrane by incorporating graphene oxide and silver-based metal-organic framework (Ag-MOF) into the TFC selective layer (Figure 12). This study showed significant improvement in membranes’ anti-biofouling and anti-fouling properties. Also, the modified TFN membrane exhibits higher hydrophilicity, water permeability, and elimination of of
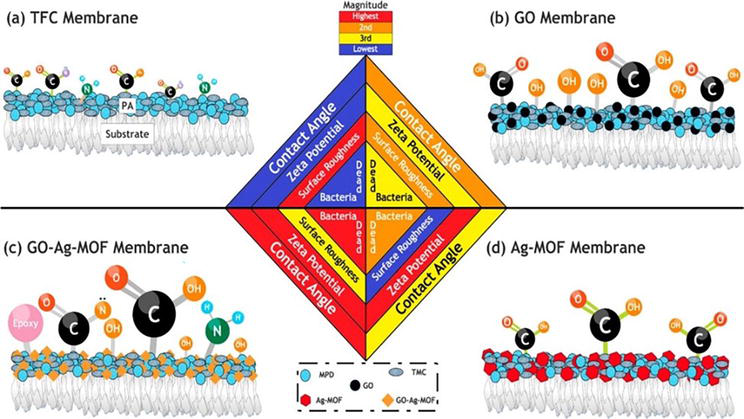
Figure 12.
Parameters contributing to anti-biofouling and anti-fouling properties of the membranes: (a) TFC, (b) GO TFN, (c) GO–Ag-MOF TFN, and (d) Ag-MOF TFN. (Source: [
4.2 Operational approaches
In addition to membrane surface modification strategies, operational parameters play a crucial role in influencing membrane performance. Factors such as temperature, applied hydraulic pressure, pH, controlled mixing, feed aeration, and the implementation of backwash cycles can collectively contribute to enhancing membrane lifespan [40]. Hydrodynamic conditions, such as cross-flow velocity and flux, significantly impact membrane fouling. High cross-flow velocity induces high shear rates, promoting particle back diffusion and reducing concentration polarization, thus minimizing fouling development. Resulting from the impact of permeate drag force on particles and bacterial attachment to the membranes, fouling occurrance was corrolated with elevated peameare flux. In addition, elevated flux also requires a higher TMP and thus, increases the hydraulic resistance of the foulant layer, which could lead to the formation of a gel layer and CP [40]. In additon, aie/gas scouring is an effective way to reduce and control membrane fouling, mainly in MF and UF systems. The mechanism of fouling minimization depends on the bubble size, where macrobubbles and air slugs are implemented in MBRs to mechanically vibrate and shake the membrane (hollo fibers), promoting local shear and limiting pore blocking and cake formation. Microbubbles are spherical and tend to spread evenly over the bulk solution while contacting the foulants to form a bubble-foulants flock ahead of the foulant-membrane contact [73]. Wu et al. examined the impact of gas slug flow on MF in an external tubular membrane reactor. The air slug flow characteristics ranged from 30 to 120 L h−1 with an interval of 30 L h−1. The shear stress was found to be 6.37 × 10−3 Pa with aeration rate of 90 L h−1 and the TMP was effectively controlled by increasing the aeration rate, with the optimal rate around 90 L h−1 [74].
4.3 Advanced fouling mitigation techniques
In recent years, novel approaches have been developed to mitigate membrane fouling, including using ultrasonic waves, heated membrane surfaces, electrically conductive membranes and more. Two examples are presented below.
Ultrasonic technology: Ultrasonic waves and energy have been used in membrane filtration systems for fouling mitigation and
Electrically Conductive Membranes (ECMs): Electrically conducting membranes are a class of specialized membranes that exhibit electrical conductivity, offering unique properties and applications across various industries. These membranes are designed to harness the benefits of electrical fields for specific purposes, including sensing, separation, and fouling mitigation. ECMs can be fabricated by coating or infusing conductive polymers like polyaniline, polypyrrole, or polythiophene. These polymers provide electrical conductivity while maintaining the flexibility and processability of the membrane. Incorporating NPs such as CNTs, graphene, or carbon black into the membrane structures enhances electrical conductivity while offering high surface area and excellent electrical properties. ECMs are highly efficient in organic fouling, biofouling, and inorganic fouling mitigation based on the externally applied potential that creates an electrostatic repulsion force between the negatively charged foulants and the membrane, reducing foulants’ attachment to the membrane. In addition, biofouling mitigation was shown to be efficient by reactive oxygen species (ROS) produced at the membrane’s surface and local pH shift [25, 79, 80, 81, 82, 83]. A recent study showed the ability to mitigate biofouling in AnMBR, where the ECM serves as a cathode and a platinum plate as the counter electrode. Bacterial inactivation was estimated under varying cathodic potentials, and biofouling formation was assessed during 48 hours of filtration. The system was efficient in fouling mitigation, mainly attributed to bacterial inactivation and biofilm mitigation from extreme local pH conditions [84]. In addition, Wang et al. [85] reported the utilization of a novel in-situ combination approach for fabricating a highly conductive carbon nanotube (CNT)/polyvinylidene fluoride (PVDF) membrane (as shown in Figure 13). The CNT/PVDF membrane had high electrical conductivity and permeability and exhibited superior anti-fouling performance in the presence of external electric fields at a 0 V/2 V-pulsed mode.
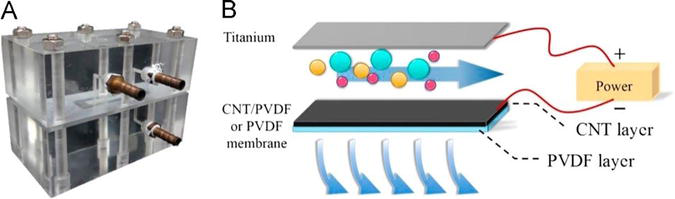
Figure 13.
(A) A photograph of the filtration cell. (B) Schematic of the electrically enhanced cross-flow filtration cell for evaluating the anti-fouling performance of the PVDF and CNT/PVDF membranes. A titanium foil was used as an anode. (Source: [
5. Summary and future outlook
Membrane technology has emerged as a crucial innovation in the field of wastewater treatment, addressing complex challenges related to water scarcity and environmental conservation. With advanced separation capabilities, this technology employs various membranes such as microfiltration, ultrafiltration, nanofiltration, and reverse osmosis to effectively eliminate a broad spectrum of contaminants. The inherent features of membrane technology, including low energy requirements, reduced reliance on chemical processes, and adaptability to diverse pollutant profiles, underscore its significance in contemporary water treatment methodologies. This technological advancement not only enhances water reuse strategies but also plays a pivotal role in promoting sustainable water resource management and environmental stewardship. However, membrane fouling in wastewater treatment poses significant limitations, characterized by the accumulation of unwanted materials on membrane surfaces, leading to decreased performance and increased operational costs. Recent scientific literature highlights diverse strategies for fouling mitigation including physical and chemical cleaning, operational optimization, and material engineering. Innovations in membrane surface modification, such as incorporating hydrophilic coatings and nano-enhanced materials, show promise in reducing fouling in addition to operational adjustments like hydraulic pressure management and implementing backwash cycles that contribute to fouling prevention and membrane longevity. These multifaceted approaches are essential for maintaining the efficiency and sustainability of membrane technologies in wastewater treatment.
The future of membrane technologies in wastewater management is defined by the integration of advanced anti-fouling strategies, innovative material science, and process engineering to significantly enhance membrane longevity and operational efficiency. Recent advancements, particularly in developing electrically conductive membranes (ECMs), demonstrate potential in addressing the persistent challenge of membrane fouling. The integration of ECMs with Joule heating capabilities, as highlighted by Liao et al. [86] serves to mitigate fouling while offering real-time fouling monitoring (Figure 14). Building on this foundation, there is an emerging inclination to explore novel materials, such as nanocomposite-based membranes exhibiting anti-fouling properties and ultra-high flux efficiency for complex separations. Furthermore, the design of hybrid processes that couple membrane bioreactors with advanced oxidation processes or bio-electrochemical systems is gaining traction. These hybrid systems aim to leverage the strengths of each component technology to enhance treatment efficiency and system stability.
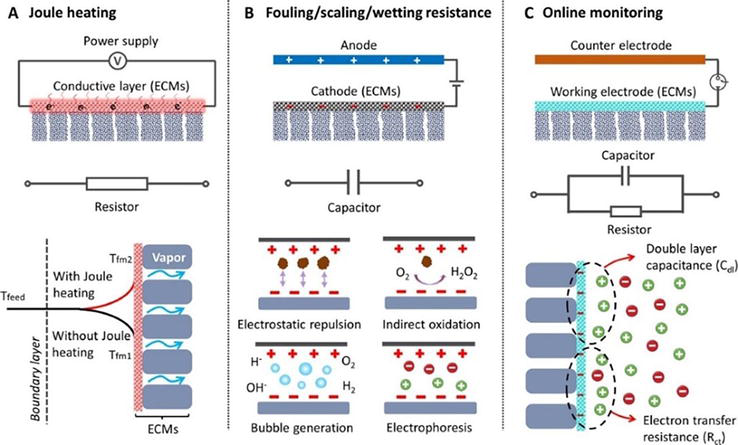
Figure 14.
Suggested future research directions. (Source: [
In summary, the future outlook for membrane technologies in sustainable wastewater management includes:
Enhancement of membrane anti-fouling properties through stable hydrophilic coatings and novel material integration.
Optimization of ECMs for improved fouling resistance, operational stability, and energy efficiency.
Development of hybrid membrane systems that synergize various treatment technologies to achieve superior performance.
Refined fabrication and characterization techniques to understand and improve membrane structure-function relationships.
Integration of real-time monitoring systems to optimize performance.
Collective efforts in these areas are poised to revolutionize wastewater treatment, pushing the boundaries of efficiency, sustainability, and resource recovery.
Acknowledgments
This work was supported by NSF-BSF funding, 2022607 (to A.R). The authors also acknowledge the postdoctoral fellowships from the Jacob Blaustein Center for Scientific Cooperation of Ben Gurion University of the Negev.
References
- 1.
Richardson K, Steffen W, Lucht W, Bendtsen J, Cornell SE, Donges JF, et al. Earth beyond six of nine planetary boundaries. Science Advances. 2023; 9 :1-17. DOI: 10.1126/sciadv.adh2458 - 2.
Calvin K, Dasgupta D, Krinner G, Mukherji A, Thorne PW, Trisos C, et al. Climate Change 2023: Synthesis Report. Geneva, Switzerland: IPCC; 2023. DOI: 10.59327/IPCC/AR6-9789291691647 - 3.
Baker RW. Membrane Technology and Applications. Newark, California: Wiley; 2004. DOI: 10.1002/0470020393 - 4.
Ahmed SF, Mehejabin F, Momtahin A, Tasannum N, Faria NT, Mofijur M, et al. Strategies to improve membrane performance in wastewater treatment. Chemosphere. 2022; 306 :135527. DOI: 10.1016/j.chemosphere.2022.135527 - 5.
Li C, Sun W, Lu Z, Ao X, Li S. Ceramic nanocomposite membranes and membrane fouling: A review. Water Research. 2020; 175 :115674. DOI: 10.1016/j.watres.2020.115674 - 6.
Deowan SA, Bouhadjar SI, Hoinkis J. Membrane Bioreactors for Water Treatment. Sawston, Cambridge: Elsevier Ltd; 2015. DOI: 10.1016/B978-1-78242-121-4.00005-8 - 7.
Younas M, Rezakazemi M. Introduction to membrane technology. In: Membrane Contactor Technology. Weinheim, Germany: Wiley; 2022. pp. 1-16. DOI: 10.1002/9783527831036.ch1 - 8.
Ezugbe EO, Rathilal S. Membrane technologies in wastewater treatment: A review. Membranes (Basel). 2020; 10 :89. DOI: 10.3390/membranes10050089 - 9.
Peters T. Membrane technology for water treatment. Chemical Engineering and Technology. 2010; 33 :1233-1240. DOI: 10.1002/ceat.201000139 - 10.
Shehata N, Egirani D, Olabi AG, Inayat A, Abdelkareem MA, Chae KJ, et al. Membrane-based water and wastewater treatment technologies: Issues, current trends, challenges, and role in achieving sustainable development goals, and circular economy. Chemosphere. 2023; 320 :137993. DOI: 10.1016/j.chemosphere.2023.137993 - 11.
Wang LK, Chen JP, Hung Y-T, Shammas NK, editors. Membrane and Desalination Technologies. Totowa, NJ: Humana Press; 2011. DOI: 10.1007/978-1-59745-278-6 - 12.
Singh R, Hankins NP. Introduction to Membrane Processes for Water Treatment. Amsterdam, Netherlands: Elsevier B.V.; 2016. DOI: 10.1016/B978-0-444-63312-5.00002-4 - 13.
Wang Q , Tang X, Liang H, Cheng W, Li G, Zhang Q , et al. Effects of filtration mode on the performance of gravity-driven membrane (GDM) filtration: Cross-flow filtration and dead-end filtration. Water (Switzerland). 2022; 14 :190. DOI: 10.3390/w14020190 - 14.
Díaz H, Azócar L, Torres A, Lopes SIC, Jeison D. Use of flocculants for increasing permeate flux in anaerobic membrane bioreactors. Water Science and Technology. 2014; 69 :2237-2242. DOI: 10.2166/wst.2014.153 - 15.
Sadr SMK, Saroj DP. Membrane Technologies for Municipal Wastewater Treatment. Sawston, Cambridge: Elsevier Ltd; 2015. DOI: 10.1016/B978-1-78242-121-4.00014-9 - 16.
Le-Clech P. Membrane bioreactors and their uses in wastewater treatments. Applied Microbiology and Biotechnology. 2010; 88 :1253-1260. DOI: 10.1007/s00253-010-2885-8 - 17.
Dezotti M, Lippel G, Bassin JP. Advanced Biological Processes for Wastewater Treatment: Emerging, Consolidated Technologies and Introduction to Molecular Techniques. Cham, Switzerland; 2017. DOI: 10.1007/978-3-319-58835-3 - 18.
Tan X, Acquah I, Liu H, Li W, Tan S. A critical review on saline wastewater treatment by membrane bioreactor (MBR) from a microbial perspective. Chemosphere. 2019; 220 :1150-1162. DOI: 10.1016/j.chemosphere.2019.01.027 - 19.
Aslam A, Khan SJ, Shahzad HMA. Anaerobic membrane bioreactors (AnMBRs) for municipal wastewater treatment- potential benefits, constraints, and future perspectives: An updated review. Science of the Total Environment. 2022; 802 :149612. DOI: 10.1016/j.scitotenv.2021.149612 - 20.
Vinardell S, Astals S, Peces M, Cardete MA, Fernández I, Mata-Alvarez J, et al. Advances in anaerobic membrane bioreactor technology for municipal wastewater treatment: A 2020 updated review. Renewable and Sustainable Energy Reviews. 2020; 130 :109936. DOI: 10.1016/j.rser.2020.109936 - 21.
Shahid MK, Kashif A, Rout PR, Aslam M, Fuwad A, Choi Y, et al. A brief review of anaerobic membrane bioreactors emphasizing recent advancements, fouling issues and future perspectives. Journal of Environmental Management. 2020; 270 :110909. DOI: 10.1016/j.jenvman.2020.110909 - 22.
Maaz M, Yasin M, Aslam M, Kumar G, Atabani AE, Idrees M, et al. Anaerobic membrane bioreactors for wastewater treatment: Novel configurations, fouling control and energy considerations. Bioresource Technology. 2019; 283 :358-372. DOI: 10.1016/j.biortech.2019.03.061 - 23.
Khan SJ, Hankins NP, Shen LC. Submerged and Attached Growth Membrane Bioreactors and Forward Osmosis Membrane Bioreactors for Wastewater Treatment. Amsterdam, Netherlands: Elsevier B.V.; 2016. DOI: 10.1016/B978-0-444-63312-5.00011-5 - 24.
Laqbaqbi M, Sanmartino JA, Khayet M, García-Payo C, Chaouch M. Fouling in membrane distillation, osmotic distillation and osmotic membrane distillation. Applied Sciences. 2017; 7 :334. DOI: 10.3390/app7040334 - 25.
Anvari A, Kekre KM, Azimi Yancheshme A, Yao Y, Ronen A. Membrane distillation of high salinity water by induction heated thermally conducting membranes. Journal of Membrane Science. 2019; 589 :117253. DOI: 10.1016/j.memsci.2019.117253 - 26.
Zhu Z, Yogev U, Goddek S, Yang F, Keesman KJ, Gross A. Carbon dynamics and energy recovery in a novel near-zero waste aquaponics system with onsite anaerobic treatment. Science of the Total Environment. 2022; 833 :155245. DOI: 10.1016/j.scitotenv.2022.155245 - 27.
Zhu Z. Nutrient Dynamics and Bioresource Recovery in Novel Zero-Waste Multi-Loop Aquaponic Systems. Netherlands: Wageningen University; 2023. DOI: 10.18174/590685 - 28.
Van der Bruggen B. Advances in Electrodialysis for Water Treatment. Sawston, Cambridge: Elsevier Ltd; 2015. DOI: 10.1016/B978-1-78242-121-4.00006-X - 29.
Kabay N, Arar Ö, Bunani S. Water Treatment by Electromembrane Processes. Amsterdam, Netherlands; 2016. DOI: 10.1016/B978-0-444-63312-5.00008-5 - 30.
Chen S, Yu J, Wang H, Yu H, Quan X. A pilot-scale coupling catalytic ozonation-membrane filtration system for recirculating aquaculture wastewater treatment. Desalination. 2015; 363 :37-43. DOI: 10.1016/j.desal.2014.09.006 - 31.
Zhou L, Tan SH, Ahmad AL, Low SC. High-flux strategy for electrospun nanofibers in membrane distillation to treat aquaculture wastewater: A review. Journal of Chemical Technology and Biotechnology. 2021; 96 :3259-3272. DOI: 10.1002/jctb.6828 - 32.
Yogev U, Gross A. Reducing environmental impact of recirculating aquaculture systems by introducing a novel microaerophilic assimilation reactor: Modeling and proof of concept. Journal of Cleaner Production. 2019; 226 :1042-1050. DOI: 10.1016/j.jclepro.2019.04.003 - 33.
Nayak S, Yogev U, Kpordzaxor Y, Zhu Z, Gur N, Gross A, et al. From fish excretions to high-protein dietary ingredient: Feeding intensively cultured barramundi (Lates calcarifer) a diet containing microbial biomass (biofloc) from effluent of an aquaculture system. Aquaculture. 2023; 562 :738780. DOI: 10.1016/j.aquaculture.2022.738780 - 34.
Li Q , Elimelech M. Organic fouling and chemical cleaning of nanofiltration membranes: Measurements and mechanisms. Environmental Science & Technology. 2004; 38 :4683-4693. DOI: 10.1021/es0354162 - 35.
Mahlangu OT, Nthunya LN, Motsa MM, Morifi E, Richards H, Mamba BB. Fouling of high pressure-driven NF and RO membranes in desalination processes: Mechanisms and implications on salt rejection. Chemical Engineering Research and Design. 2023; 199 :268-295. DOI: 10.1016/j.cherd.2023.09.037 - 36.
Guo W, Ngo HH, Li J. A mini-review on membrane fouling. Bioresource Technology. 2012; 122 :27-34. DOI: 10.1016/j.biortech.2012.04.089 - 37.
Yiantsios SG, Karabelas AJ. The effect of colloid stability on membrane fouling. Desalination. 1998; 118 :143-152. DOI: 10.1016/S0011-9164(98)00110-6 - 38.
Huang H, Spinette R, O’Melia CR. Direct-flow microfiltration of aquasols. I. Impacts of particle stabilities and size. Journal of Membrane Science. 2008; 314 :90-100. DOI: 10.1016/j.memsci.2008.01.040 - 39.
Lim AL, Bai R. Membrane fouling and cleaning in microfiltration of activated sludge wastewater. Journal of Membrane Science. 2003; 216 :279-290. DOI: 10.1016/S0376-7388(03)00083-8 - 40.
Goh PS, Lau WJ, Othman MHD, Ismail AF. Membrane fouling in desalination and its mitigation strategies. Desalination. 2018; 425 :130-155. DOI: 10.1016/j.desal.2017.10.018 - 41.
Sisay EJ, Al-Tayawi AN, László Z, Kertész S. Recent advances in organic fouling control and mitigation strategies in membrane separation processes. A Review. Sustainability. 2023; 15 :13389. DOI: 10.3390/su151813389 - 42.
Firouzjaei MD, Seyedpour SF, Aktij SA, Giagnorio M, Bazrafshan N, Mollahosseini A, et al. Recent advances in functionalized polymer membranes for biofouling control and mitigation in forward osmosis. Journal of Membrane Science. 2020; 596 :117604. DOI: 10.1016/j.memsci.2019.117604 - 43.
Deng L, Guo W, Ngo HH, Zhang H, Wang J, Li J, et al. Biofouling and control approaches in membrane bioreactors. Bioresource Technology. 2016; 221 :656-665. DOI: 10.1016/j.biortech.2016.09.105 - 44.
Ke X, Hongqiang R, Lili D, Jinju G, Tingting Z. A review of membrane fouling in municipal secondary effluent reclamation. Environmental Science and Pollution Research. 2013; 20 :771-777. DOI: 10.1007/s11356-012-1147-y - 45.
Bui X-T, Chiemchaisri C, Fujioka T, Varjani S. Biofouling Detection on Reverse Osmosis Membranes BT - Water and Wastewater Treatment Technologies. Singapore: Springer; 2019. DOI: 10.1007/978-981-13-3259-3 - 46.
Lopez-Vazquez CM, Brdjanovic D, Volcke E, van Loosdrecht MCM, Wu D, Chen G. Biological Wastewater Treatment - Examples and Exercises. London, UK: IWA; 2023. DOI: 10.2166/9781789062304 - 47.
Asadollahi M, Bastani D, Musavi SA. Enhancement of surface properties and performance of reverse osmosis membranes after surface modification: A review. Desalination. 2017; 420 :330-383. DOI: 10.1016/j.desal.2017.05.027 - 48.
Choi H, Park J, Tak T, Kwon YN. Surface modification of seawater reverse osmosis (SWRO) membrane using methyl methacrylate-hydroxy poly(oxyethylene) methacrylate (MMA-HPOEM) comb-polymer and its performance. Desalination. 2012; 291 :1-7. DOI: 10.1016/j.desal.2012.01.018 - 49.
Ishigami T, Amano K, Fujii A, Ohmukai Y, Kamio E, Maruyama T, et al. Fouling reduction of reverse osmosis membrane by surface modification via layer-by-layer assembly. Separation and Purification Technology. 2012; 99 :1-7. DOI: 10.1016/j.seppur.2012.08.002 - 50.
Kim JS, Kim DH, Hwang DK. Efficiency enhancement of bifacial Cu2ZnSnSe4 thin-film solar cells on indium tin oxide glass substrates by suppressing In-Sn diffusion with Mo interlayer. Journal of Power Sources. 2018; 400 :9-15. DOI: 10.1016/j.jpowsour.2018.08.001 - 51.
Yu S, Liu X, Liu J, Wu D, Liu M, Gao C. Surface modification of thin-film composite polyamide reverse osmosis membranes with thermo-responsive polymer (TRP) for improved fouling resistance and cleaning efficiency. Separation and Purification Technology. 2011; 76 :283-291. DOI: 10.1016/j.seppur.2010.10.017 - 52.
Lin B, Tan H, Liu W, Gao C, Pan Q. Preparation of a novel zwitterionic striped surface thin-film composite nanofiltration membrane with excellent salt separation performance and anti-fouling property. RSC Advances. 2020; 10 :16168-16178. DOI: 10.1039/d0ra00480d - 53.
Song F, Tu C, Meng F, Wang L, Xia L, Zhao S. Engineering a novel zwitterionic polyurethane nanofiltration membrane with enhanced fouling resistance for water reclamation. Desalination. 2023; 551 :116440. DOI: 10.1016/j.desal.2023.116440 - 54.
Xia L, Song F, Meng F, Wang L, Zhao S. Engineering anti-fouling nanofiltration membranes using amino-quinone networks-phytic acid pseudo zwitterionic clusters for water treatment. ACS EST Water. 2023; 3 :1923-1934. DOI: 10.1021/acsestwater.3c00015 - 55.
Chi M, Zheng P, Wei M, Zhu A, Zhong L, Zhang Q , et al. Polyamide composite nanofiltration membrane modified by nanoporous TiO2 interlayer for enhanced water permeability. Journal of Industrial and Engineering Chemistry. 2022; 115 :230-240. DOI: 10.1016/j.jiec.2022.08.004 - 56.
Gao S, Zhu Y, Gong Y, Wang Z, Fang W, Jin J. Ultrathin polyamide Nanofiltration membrane fabricated on brush-painted single-walled carbon nanotube network support for ion sieving. ACS Nano. 2019; 13 :5278-5290. DOI: 10.1021/acsnano.8b09761 - 57.
Wang A, Xu H, Fu J, Lin T, Ma J, Ding M, et al. Enhanced high-salinity brines treatment using polyamide nanofiltration membrane with tunable interlayered MXene channel. Science of the Total Environment. 2023; 856 :158434. DOI: 10.1016/j.scitotenv.2022.158434 - 58.
Zhao DL, Zhao Q , Lin H, Chen SB, Chung TS. Pressure-assisted polydopamine modification of thin-film composite reverse osmosis membranes for enhanced desalination and anti-fouling performance. Desalination. 2022; 530 :115671. DOI: 10.1016/j.desal.2022.115671 - 59.
Jee KY, Shin DH, Lee YT. Surface modification of polyamide RO membrane for improved fouling resistance. Desalination. 2016; 394 :131-137. DOI: 10.1016/j.desal.2016.05.013 - 60.
Xu L, Pang Z, Yu H, Guo M, Yan X, Jiang X, et al. Anti-fouling loose nanofiltration membranes prepared via the fast codeposition of capsaicin-mimic/polydopamine for efficient dye/salt separation. Desalination. 2023; 565 :116809. DOI: 10.1016/j.desal.2023.116809 - 61.
Qiu Z, Chen J, Dai R, Wang Z. Modification of ultrafiltration membrane with antibacterial agent intercalated layered nanosheets: Toward superior antibiofouling performance for water treatment. Water Research. 2022; 219 :118539. DOI: 10.1016/j.watres.2022.118539 - 62.
Huyen DTT, Ray SS, Kwon YN. Zwitterionic material for construction of an antifouling polyamide thin film composite membrane. Desalination. 2024; 572 :117118. DOI: 10.1016/j.desal.2023.117118 - 63.
Deng L, Li S, Qin Y, Zhang L, Chen H, Chang Z, et al. Fabrication of anti-fouling thin-film composite nanofiltration membrane via surface grafting of polyethyleneimine followed by zwitterionic modification. Journal of Membrane Science. 2021; 619 :118564. DOI: 10.1016/j.memsci.2020.118564 - 64.
Zhang X, He F, Yang Z, Xie M, Ren H, Wu D, et al. Engineering polyamide nanofiltration membrane with bifunctional terpolymer brushes for anti-fouling and antimicrobial properties. Desalination. 2023; 558 :116642. DOI: 10.1016/j.desal.2023.116642 - 65.
Daer S, Kharraz J, Giwa A, Hasan SW. Recent applications of nanomaterials in water desalination: A critical review and future opportunities. Desalination. 2015; 367 :37-48. DOI: 10.1016/j.desal.2015.03.030 - 66.
Xie T, Li F, Chen K, Zhao S, Chen Y, Sun H, et al. Fabrication of novel thin-film nanocomposite polyamide membrane by the interlayer approach: A review. Desalination. 2023; 554 :116509. DOI: 10.1016/j.desal.2023.116509 - 67.
Zhao S, Liao Z, Fane A, Li J, Tang C, Zheng C, et al. Engineering anti-fouling reverse osmosis membranes: A review. Desalination. 2021; 499 :114857. DOI: 10.1016/j.desal.2020.114857 - 68.
Ong CS, Goh PS, Lau WJ, Misdan N, Ismail AF. Nanomaterials for biofouling and scaling mitigation of thin film composite membrane: A review. Desalination. 2016; 393 :2-15. DOI: 10.1016/j.desal.2016.01.007 - 69.
Noor U, Farid MF, Sharif A, Saleem A, Nabi Z, Mughal MF, et al. Functionalized carbon 1D/2D nanomaterials for effective water desalination: Synthesis, applications and cost issues. An overview. Desalination. 2024; 571 :117086. DOI: 10.1016/j.desal.2023.117086 - 70.
Yin J, Deng B. Polymer-matrix nanocomposite membranes for water treatment. Journal of Membrane Science. 2015; 479 :256-275. DOI: 10.1016/j.memsci.2014.11.019 - 71.
Firouzjaei MD, Shamsabadi AA, Aktij SA, Seyedpour SF, Sharifian M, Rahimpour A, et al. Exploiting synergetic effects of graphene oxide and a silver-based metal-organic framework to enhance anti-fouling and anti-biofouling properties of thin-film nanocomposite membranes. ACS Applied Materials & Interfaces. 2018; 10 :42967-42978. DOI: 10.1021/acsami.8b12714 - 72.
Suresh D, Goh PS, Ismail AF, Mansur SB, Wong KC, Asraf MH, et al. Complexation of tannic acid/silver nanoparticles on polyamide thin film composite reverse osmosis membrane for enhanced chlorine resistance and anti-biofouling properties. Desalination. 2022; 543 :116107. DOI: 10.1016/j.desal.2022.116107 - 73.
Levitsky I, Tavor D, Gitis V. Micro and nanobubbles in water and wastewater treatment: A state-of-the-art review. Journal of Water Process Engineering. 2022; 47 :102688. DOI: 10.1016/j.jwpe.2022.102688 - 74.
Wu Y, Wang J, Zhang H, Ngo HH, Guo W, Zhang N. The impact of gas slug flow on microfiltration performance in an airlift external loop tubular membrane reactor. RSC Advances. 2016; 6 :109067-109075. DOI: 10.1039/c6ra19903h - 75.
Qasim M, Darwish NN, Mhiyo S, Darwish NA, Hilal N. The use of ultrasound to mitigate membrane fouling in desalination and water treatment. Desalination. 2018; 443 :143-164. DOI: 10.1016/j.desal.2018.04.007 - 76.
Naji O, Al-juboori RA, Khan A, Yadav S, Altaee A, Alpatova A, et al. Ultrasound-assisted membrane technologies for fouling control and performance improvement: A review. Journal of Water Process Engineering. 2021; 43 :102268. DOI: 10.1016/j.jwpe.2021.102268 - 77.
Camara HWD, Doan H, Lohi A. In-situ ultrasound-assisted control of polymeric membrane fouling. Ultrasonics. 2020;108 :106206. DOI: 10.1016/j.ultras.2020.106206 - 78.
Ehsani M, Doan H, Lohi A, Zhu N, Abdelrasoul A. Experimental and statistical study of an in-situ ultrasound (US)-assisted ultrafiltration process: Application to fouling control in skimmed milk filtration. Journal of Water Process Engineering. 2022;49 :103171. DOI: 10.1016/j.jwpe.2022.103171 - 79.
Duan W, Chen G, Chen C, Sanghvi R, Iddya A, Walker S, et al. Electrochemical removal of hexavalent chromium using electrically conducting carbon nanotube/polymer composite ultrafiltration membranes. Journal of Membrane Science. 2017; 531 :160-171. DOI: 10.1016/j.memsci.2017.02.050 - 80.
Duan W, Ronen A, Walker S, Jassby D. Polyaniline-coated carbon nanotube ultrafiltration membranes: Enhanced anodic stability for in situ cleaning and electro-oxidation processes. ACS Applied Materials & Interfaces. 2016; 8 :22574-22584. DOI: 10.1021/acsami.6b07196 - 81.
Ronen A, Walker SL, Jassby D. Electroconductive and electroresponsive membranes for water treatment. Reviews in Chemical Engineering. 2016; 32 :533-550. DOI: 10.1515/revce-2015-0060 - 82.
Ronen A, Duan W, Wheeldon I, Walker S, Jassby D. Microbial attachment inhibition through Low-voltage electrochemical reactions on electrically conducting membranes. Environmental Science & Technology. 2015; 49 :12741-12750. DOI: 10.1021/acs.est.5b01281 - 83.
Thamaraiselvan C, Ronen A, Lerman S, Balaish M, Ein-Eli Y, Dosoretz CG. Low voltage electric potential as a driving force to hinder biofouling in self-supporting carbon nanotube membranes. Water Research. 2018; 129 :143-153. DOI: 10.1016/j.watres.2017.11.004 - 84.
Qi S, Grossman AD, Ronen A, Bernstein R. Low-biofouling anaerobic electro-conductive membrane bioreactor: The role of pH changes in bacterial inactivation and biofouling mitigation. Journal of Membrane Science. 2022; 662 :120960. DOI: 10.1016/j.memsci.2022.120960 - 85.
Wang S, Liang S, Liang P, Zhang X, Sun J, Wu S, et al. In-situ combined dual-layer CNT/PVDF membrane for electrically-enhanced fouling resistance. Journal of Membrane Science. 2015; 491 :37-44. DOI: 10.1016/j.memsci.2015.05.014 - 86.
Liao X, Lim YJ, Khayet M, Liao Y, Yao L, Zhao Y, et al. Applications of electrically conductive membranes in water treatment via membrane distillation: Joule heating, membrane fouling/scaling/wetting mitigation and monitoring. Water Research. 2023; 244 :120511. DOI: 10.1016/j.watres.2023.120511