Abstract
Despite concerns about diminishing fossil fuels and the imperative for renewable alternatives, lignin, Earth’s most abundant aromatic biopolymer, remains largely underutilized. This chapter explores the immense potential of lignin biorefinery to address energy demands, promote economic growth, and adhere to sustainable development principles. However, intricate structure, harsh odor, and toxicity hinder its valorization. To address these issues, examining emerging biochemical strategies, including thermochemical and enzymatic depolymerization and physical techniques, have emerged as promising avenues for converting lignin into valuable biofuels and chemical compounds. By highlighting innovative approaches and technologies, it emphasizes the pivotal role of lignin in driving biorefineries toward low-emission processes, yielding a diverse spectrum of bio-products. This chapter aims to contribute to the ongoing discourse on sustainable and eco-friendly biorefinery practices of lignin valorization.
Keywords
- lignin
- depolymerization
- biorefinery
- sustainable valorization
- biofuels
- bioproducts
1. Introduction
In recent years, growing concerns about the depletion of fossil fuels have ignited a quest for sustainable and renewable alternatives to meet the escalating global energy demands [1, 2, 3]. Amidst this backdrop, lignin, the most abundant aromatic biopolymer on Earth, emerges as a promising solution, representing an impressive reservoir of approximately 300 billion metric tons [4, 5]. Leveraging lignin for producing biofuels and diverse chemicals through biorefinery processes holds the potential to not only alleviate the strain on diminishing fossil fuel resources but also propel economic growth while adhering to principles of sustainable development. However, the realization of lignin’s full potential faces formidable challenges. A significant portion of lignin is relegated to combustion, a practice that fails to harness its intrinsic value [6]. This predicament is primarily attributed to lignin’s intricate and rigid structures, pungent odor, and toxicity to certain organisms, rendering sustainable valorization a complex endeavor [7]. As shown in Figure 1, biochemical strategies, such as thermochemical coupled with physical techniques and microbial enzymatic depolymerization, have emerged as promising avenues for converting lignin into value-added compounds [8].
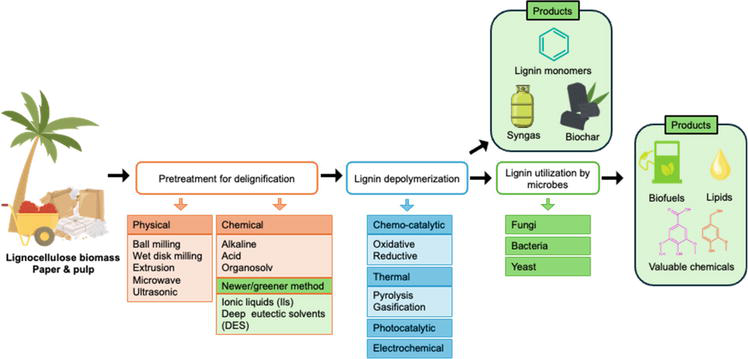
Figure 1.
Overview of lignin valorization for biorefinery application.
This book chapter endeavors to shed light on the existing challenges and breakthroughs in the sustainable valorization of lignin for biorefinery applications. By delving into innovative approaches and technologies, the chapter aims to underscore the pivotal role of lignin in steering future biorefineries toward low-emission processes, yielding a spectrum of valuable biofuels and chemical compounds. This chapter aspires to contribute to the ongoing discourse on lignin utilization for sustainable and eco-friendly biorefinery practices by comprehensively exploring the current landscape.
2. Lignin as a valorization material
2.1 Structure and properties
The intricate structure of lignin as a natural macromolecule comprises methoxy groups, phenolic hydroxyl groups, and a few terminal aldehyde groups. These functional groups are attached to a complex network of phenylpropanoid units, the fundamental building blocks of lignin [9]. The three main types of phenylpropanoids in lignin are p-hydroxyphenyl (H), guaiacyl (G), and syringyl (S), each distinguished by the degree of methoxylation on their aromatic rings (Figure 2) [10]. The aromatic rings of phenylpropanoid units, abundant in lignin, impart antioxidant activity [11]. These monolignols undergo random coupling through various linkages, and this coupling process results in a diverse array of C-O-C and C-C linkages. The linkages include α-O-4, β-O-4, 4-O-5 (C-O-C) and β-β, β-1, β-5, 5-5 (C-C) [12, 13]. Primarily, β-O-4 forms the three-dimensional structure of lignin, accounting for up to 50% of all bonds [14, 15]. Unlike cellulose and hemicellulose, where the biopolymer is readily decomposed into sugars [16], the highly polymerized lignin exhibits a rigid structure that resists chemical pretreatment [17]. Cleaving the β-O-4 linkage has been a crucial target for lignin depolymerization strategies. The source of biomass strongly influences the structure and composition of lignin. For instance, softwood lignin contains a high proportion of G units (80–90%), hardwood lignin contains a mixture of G (25–50%) and S (50–70%) units, and grass lignin comprises a mix of G (25–50%), H (10–25%), and S (25–50%) aromatic units [14, 18]. Consistently, S/G-rich lignin is preferred for maximizing monomer yields in biorefining applications due to its ability to reduce the formation of inactive C-C bonds, leading to undesired condensed lignin during lignin depolymerization. High G and low S content in lignin could lead to a surge in β-O-4 linkages, creating readily recombining monomers during fractionation [19, 20]. Understanding lignin’s structure and identifying its native linkages are essential for its efficient valorization into renewable fuels, materials, or chemicals (Figure 3).
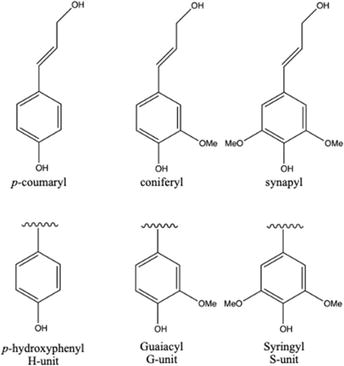
Figure 2.
Relative structures of group units of lignin.
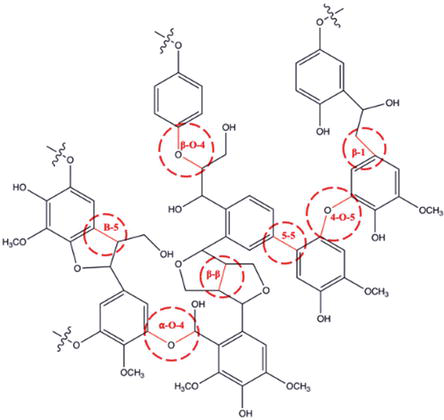
Figure 3.
Lignin polymer structure and its main linkages.
2.2 Lignin characterization
To effectively utilize the lignin from the abundant type of lignocellulosic biomass (LB), it is essential to understand its intricate structure and properties. The various pretreatment methods employed for lignin extraction can drastically alter its structure, composition, and properties. Several factors, including the origin of the lignocellulosic biomass, the pretreatment process, and the specific isolation and purification techniques used, influence the extent of these modifications. Therefore, to effectively valorize the extracted lignin into valuable aromatic compounds, it is crucial to understand the precise changes induced in its physicochemical properties through meticulous characterization techniques.
Various characterization techniques play a vital role in unveiling lignin’s secrets. Two prominent techniques are gel permeation chromatography (GPC) and Fourier transform infrared spectroscopy (FTIR). GPC measures molecular weight distribution, revealing how pretreatment methods like THF-based extraction affect lignin’s size [21]. FTIR provides information on functional groups present in the structure. The presence of specific bands, such as those corresponding to aromatic O∙H and methoxy groups, confirms the integrity of the lignin structure [22]. Determining thermal stability is crucial for various applications. Thermogravimetric analysis (TGA) and differential scanning calorimetry (DSC) offer valuable insights. TGA reveals the weight loss pattern as temperature increases, indicating the presence of different chemical bonds and their decomposition temperatures [23]. DSC helps determine glass transition temperature (Tg) and heat of pyrolysis. Higher Tg values suggest better thermal stability, with lignin boasting higher Tg compared to synthetic polymers due to its complex structure [24]. NMR spectroscopy shines in unraveling the detailed structure of lignin. 1H NMR provides information about different functional groups based on proton signals. 13C NMR offers better resolution and facilitates the quantification of specific linkages like β-O-4 and β-β bonds [25]. 31P NMR allows rapid quantification of hydroxyl groups using phosphorylation. 2D HSQC NMR or 2D 1H-13C heteronuclear single quantum coherence NMR provides high resolution, enabling the identification of different monolignol units, their ratios, and the relative abundance of interunit linkages [26]. By combining the information gleaned from these diverse characterization techniques, researchers can profoundly understand lignin’s structure and properties. This knowledge paves the way for its effective utilization in various applications, ranging from biofuels and bioplastics to sustainable materials and pharmaceuticals.
3. Pretreatment for lignocellulosic biomass delignification
3.1 The power of physical pretreatment
Physical pretreatment is the essential first step in unlocking the potential of lignocellulosic biomass for biofuel production. By reducing particle size, increasing surface area, and altering crystallinity, it paves the way for efficient subsequent processes like enzymatic hydrolysis and fermentation [27]. While energy consumption remains a challenge, with some methods requiring significant power, the advantages of physical pretreatment are undeniable. Its eco-friendly nature, minimal toxic byproduct generation, and effectiveness on a wide variety of feedstocks make it a crucial tool in the biofuel toolbox [28]. Ongoing research in optimizing energy efficiency, like the use of wet disk milling, promises to further enhance the sustainability and cost-effectiveness of this essential pretreatment technique. Milling is the most prevalent physical pretreatment method and dominates the scene thanks to its versatility and effectiveness [29]. However, innovative approaches like microwave irradiation and extrusion are emerging as powerful alternatives. Microwave pretreatment utilizes the unique properties of electromagnetic waves to disrupt the lignocellulosic structure, often achieving high sugar yields in a short timeframe [30]. Extrusion, combined with high temperature and shear forces, effectively breaks down the recalcitrance of lignocellulose, leading to enhanced enzymatic digestibility [31]. Both methods offer distinct advantages, with microwave pretreatment boosting energy efficiency and reduced inhibitor formation, while extrusion excels in its ability to handle diverse biomass types and produce high-quality sugars.
Ultrasonication, harnessing the power of sound waves, stands out as a promising frontier in physical pretreatment. By creating cavitation bubbles that collapse and generate reactive radicals (H and OH) in the medium to disrupt the lignocellulosic structure, it efficiently increases surface area and digestibility [32]. While factors like frequency, duration, and power need careful optimization to achieve optimal results, the potential benefits are substantial. Ultrasonication can significantly reduce hydrolysis times, leading to faster biofuel production. Its ability to pretreat various materials, including sugar beet shreds and filter paper, further underscores its versatility [33]. Although energy consumption remains a concern, ongoing research in optimizing parameters and integrating ultrasonication with other processes holds great promise for unlocking the full potential of this innovative pretreatment method.
3.2 Chemical pretreatment for enhanced material processing
3.2.1 Alkaline pretreatment
Solubilizing lignin, the recalcitrant barrier shields cellulose and hemicellulose from enzymatic digestion. The workhorses of the alkali pretreatment are sodium, potassium, calcium, and ammonium hydroxides. Sodium hydroxide (NaOH) leads the charge, cleaving the bonds between lignin and other polymers and releasing them into the solution [34]. This exposes the cellulose for enzymes to feast on and swells its structure, reducing crystallinity and increasing surface area for digestion. As if that is not enough, alkali pretreatment removes acetyl groups and uronic acids from hemicellulose, further enhancing enzymatic accessibility [35]. The evidence for the prowess of alkali pretreatment is overwhelming. Sugarcane bagasse, optimized with alkali pretreatment, yielded 82.2% more methane [36]. Rice straw shows a similar trend, treated with 1% NaOH for 3 h at room temperature, and saw a 34% boost in methane yield [37]. Ammonia-based pretreatments are also gaining traction due to their eco-friendly nature and ease of recovery. Studies on hardwood species and energy cane bagasse demonstrate their potential for sugar production [38]. Despite its effectiveness, alkali pretreatment has its Achilles’ heel: alkali recovery. This adds complexity and cost to the process. Additionally, while effective for low-lignin biomass like herbaceous crops and agricultural residues, hardwoods remain a tougher nut to crack. In the end, alkali pretreatment remains a powerful tool for unlocking the potential of lignocellulosic biomass. Addressing its recovery challenges and tailoring it for diverse biomass types will further strengthen its position as a key player in the bioeconomy revolution.
3.2.2 Acid pretreatment
The acid pretreatment process utilizes the acidic properties of various chemicals to break down the complex structure of lignocellulosic biomass, primarily targeting and break the glycosidic bonds via SN1 reaction that hold cellulose and hemicellulose together [39]. Both inorganic and organic acids are employed, each offering distinct advantages and disadvantages. Mineral acids like sulfuric, phosphoric, nitric, and hydrochloric are commonly used for their effectiveness and low cost. However, they can be highly corrosive and generate inhibitory byproducts during pretreatment [40]. Organic acids like formic, maleic, and oxalic acid are gentler on the biomass and produce fewer inhibitors, but their efficacy might be lower, and their cost can be higher [41]. Acid pretreatments can be tailored depending on the specific LB and desired outcome. Concentrated acids at range 30–70% at low temperatures lower than 100°C achieve rapid sugar conversion but are associated with high costs and environmental concerns. Dilute acids at range 0.1–10% at high temperatures from100 to 250°C are more economical and environmentally friendly but require longer processing times. One major challenge of acid pretreatment is the formation of inhibitory compounds like furfurals and phenolic acids, which hinder subsequent enzymatic hydrolysis and fermentation [42]. Chemical and biological detoxification methods are employed to mitigate this issue. Chemical approaches involve using adsorbents like activated charcoal or enzymatic treatments [43]. Biological methods utilize microbes to convert inhibitors into less harmful substances [44]. The optimal acid and pretreatment conditions depend on several factors, including the type of LB, desired sugar yield, inhibitor formation, and cost-effectiveness. Studies have shown that dilute sulfuric acid is often the most effective and economical choice for pretreating various LBs [45]. However, research on organic acids, surfactants, and targeted pretreatments of specific LB components holds promise for further improvements in efficiency and sustainability [45, 46]. Acid pretreatment plays a crucial role in unlocking the potential of LBs for biofuel production. Careful optimization of acid type, pretreatment conditions, and downstream detoxification processes is essential for maximizing sugar yield and achieving a cost-effective and environmentally friendly biofuel production system.
The quest for sustainable biofuels from lignocellulosic biomass hinges on efficient pretreatment, breaking down the complex structure for enzymatic conversion. While traditional methods often rely on harsh chemicals and energy-intensive processes, three promising alternatives emerge, including ionic liquids (ILs), organosolv, and deep eutectic solvents (DES). Under acidic conditions, benzyl cation, an active intermediate of lignin, is produced during the separation process, and its condensation will deepen the color of lignin [47]. A large number of literatures have reported that alcohols with high boiling points, such as diols or triols, can effectively protect the benzyl groups of lignin in the acid-catalyzed treatment of hardwood and herbaceous lignocellulose, thus effectively separating light-colored and low condensation lignin for further application [48, 49, 50]. So that why, recently, DES was synthesized with choline chloride, high-boiling-point alcohol (1,4-butanediol glycerin, propylene glycol, and ethylene glycol), and Lewis acid (AlCl3, FeCl3, ZnCl2, and CuCl2) for acid pretreatment of softwood to extract lignin.
3.2.3 Ionic liquids
Introduced in 2002, ILs offer fascinating possibilities. These designer solvents, with custom-tailored cations and anions, can dissolve cellulose and lignin, enhancing enzymatic digestibility [47]. Their adjustable properties, like low volatility and reusability, make them “green” champion candidate options. Imidazolium-based ILs were effective in improving sugar yield from LBs up to 97.7%. However, cost and potential enzyme toxicity remain hurdles [48, 49]. On the other hand, low-cost triethylammonium hydrogen sulfate, showcasing high lignin (up to 85%) and hemicellulose solubilization with efficient recovery, with a 75% deduction pretreatment cost, offers promising insights for future development [50]. Acid catalysts, such as H3PO4 and H2SO4, are commonly used in ionic liquids to break down the β-O-4 linkage (Figure 4) [51, 52].

Figure 4.
Oxidation depolymerization of lignin via ionic liquids.
3.2.4 Organosolv
This method utilizes organic solvents like acetic acid, acetone, dimethyl sulfoxide (DMSO), ethanol, formic acid, methanol, or tetrahydrofuran mixed with inorganic acid catalysts to break down lignocellulose biomass internal bonds (lignin and/or hemicellulose), leaving behind pure cellulose [53]. Its advantages include easy solvent recovery and high-quality lignin byproduct generation. However, the process is energy-intensive, and the high cost and volatility of organic solvents pose concerns. Research on efficient solvent recovery and exploration of less volatile alternatives is crucial for organosolv’s long-term success. Ethanol organosolv mixed with 2-naphthol-7-sulfonate improved the lignin selective expelling with an 85% cellulose hydrolysis yield. High selectivity for lignin removal can be beneficial for lignin polymer utilization [54].
3.2.5 Deep eutectic solvents: a new Dawn in pretreatment
DES, a newcomer in the field, offers a compelling alternative. These mixtures of two or three components, often interlinked by hydrogen bonds, are liquid at low temperatures and possess properties similar to ILs. Chlorine chloride in the lactic acid by the ratio of 1:10, at 130°C for 4 h, successfully boosted the lignin recovery up to 72.3% with high purity at 92.5% from the lignin removal rate of 81.2% [55]. Their key advantages lie in their lower cost, biodegradability, and ease of synthesis. These advantages make DES a promising contender for sustainable LB processing [56].
3.2.6 The road ahead: a balancing act
Each method presents unique strengths and weaknesses. ILs offer tailored properties and high efficiency but face cost and toxicity challenges. Organosolv, a familiar option, requires efficient solvent recovery and cost reduction. DES, the new kid on the block, holds promise due to its sustainability, but further research is needed to optimize its performance. Ultimately, the ideal pretreatment method for LB will strike a delicate balance between efficiency, cost, and environmental impact. Continued research and development in these areas will pave the way for a future where sustainable biofuels from LBs become a reality.
4. Lignin depolymerization
4.1 Chemo-catalytic depolymerization
The burgeoning field of chemo-catalytic depolymerization offers a transformative approach to resource recovery by breaking down complex polymers into their valuable monomeric constituents. This exciting technology transcends the limitations of traditional depolymerization methods, often plagued by harsh conditions, incomplete conversion, and undesired byproducts. By harnessing the synergistic interplay of chemical reagents and tailored catalysts, chemo-catalytic depolymerization unlocks a treasure trove of possibilities [57]. Imagine lignin waste from the bioethanol or paper industries being deconstructed at the molecular level, its hydrocarbon chains cleaved with exquisite precision to yield pristine monomers. These recovered building blocks can then be seamlessly reintegrated into novel polymer production, forming a closed-loop system that dramatically reduces reliance on fossil fuels and minimizes environmental footprint.
The chemo-catalytic depolymerization lies in its exquisite selectivity. Catalysts act as molecular matchmakers, guiding the depolymerization process toward desired products while minimizing unwanted side reactions [58]. This level of control paves the way for the recovery of not just bulk monomers, but also specific high-value chemicals trapped within complex polymers. This opens doors to exciting new avenues in fields ranging from green chemistry to biorefinery. However, challenges remain. Designing efficient and cost-effective catalysts for diverse polymer feedstocks is an ongoing pursuit. Additionally, optimizing reaction conditions and overcoming potential scalability hurdles are crucial steps toward translating this promising technology into real-world applications. Despite these challenges, the potential of chemo-catalytic depolymerization to revolutionize resource utilization is undeniable. By harnessing the power of chemistry and catalysis, researchers can unlock a future where lignin waste becomes a valuable resource, paving the way for a more sustainable and circular economy. Oxidative and reduction depolymerization are well-known chemo-catalytic depolymerizations for lignin valorizations.
4.1.1 Oxidative depolymerization
The study of lignin oxidation mechanisms is crucial for optimizing its depolymerization into valuable chemicals. Oxidation depolymerization of lignin from LB is favorable for producing various valuable aromatic compounds, including aromatic derivative monomeric aldehydes to aromatic acids, and carboxylic acids. Lignin-derivative aldehydes produced by the oxidation process, namely,
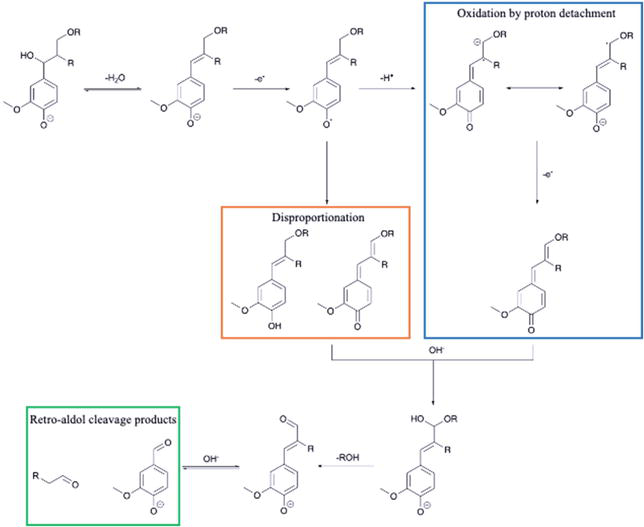
Figure 5.
Reaction mechanism for vanillin (model) via alkaline oxidative of lignin.
The alkaline solvent is commonly used in oxidative depolymerization. In alkaline environments, phenolic compounds readily lose their protons, transforming into negatively charged phenolate anions. This deprotonation sets the stage for further oxidation, primarily through the formation of phenoxy radicals. These radicals can also arise indirectly, as peroxy radicals generated from dioxygen radicals react with phenolate anions, creating peroxide anions. These highly reactive peroxide anions then engage in an intramolecular nucleophilic attack on the carbon atom of the phenolate species, ultimately leading to the formation of dioxetane intermediates, key players in the subsequent oxidative degradation of lignin [66].
In the nitrobenzene oxidation, the reaction begins with the activation of nitrobenzene by a strong base, typically sodium hydroxide (NaOH), to form a nitrobenzene radical anion. The nitrobenzene radical anion then transfers an electron to a lignin phenolate anion, a negatively charged aromatic ring present in the lignin structure. This oxidizes the phenolate anion to a phenoxyl radical, and the nitrobenzene radical anion is converted back to neutral nitrobenzene. The phenoxyl radical undergoes a series of cleavage and rearrangement reactions, depending on the specific lignin structure. Common pathways include β-O-4 cleavage (cleavage of the Cα-Cβ bond adjacent to the phenolic oxygen, leading to fragments containing aldehyde groups), α-O-4 cleavage (cleavage of the Cβ-Cγ bond, also resulting in aldehyde-containing fragments), and side-chain cleavage, resulting in fragments containing aldehyde groups. These aldehyde fragments can further undergo oxidation and decarboxylation to form the final products, namely vanillin (from guaiacyl units in lignin) and syringaldehyde (from syringyl units in lignin). While most reaction pathways favor aldehyde formation, excessive oxidation (overoxidation) can break down the aldehyde chain further, leading to the corresponding aromatic acids [59, 67, 68]. Some intermediate hydroxy acids formed during lignin cleavage can also undergo decarboxylation to yield aromatic acids, and certain side reactions with intermediates can also form aromatic acids as minor products. Compared to oxygen, nitrobenzene showed significant results for better production of lignin monomeric aldehydes and acids. In the 10% of NaOH, nitrobenzene significantly outperformed oxygen oxidation [69]. The influence of Cu (metal oxide) in lanthanum cobaltite (LaCoO3) catalysts on product yield. Increasing Cu content generates more active sites, boosting p-hydroxybenzaldehyde, vanillin, and syringaldehyde production [70]. The use of polyoxometalate catalysts. While H3PMo12O40 in N2 yields low products due to catalyst reduction, adding methanol as a scavenger improves conversion by preventing re-polymerization. Their study with 80% methanolic water and recycled catalysts shows up to 7 wt% combined vanillin and methyl vanillate yield [71]. In terms of specific oxidation, reactions can be employed in the production of biopolymers, such as adhesives, films, and coatings. The specific oxidation of biopolymers can be achieved using various oxidizing agents, such as periodate, hypochlorite, or nitroxyl radicals, under controlled conditions to target-specific functional groups within the polymer structure (like -OH, C∙C cleavages). One prominent example is the oxidation of polysaccharides like starch to produce biopolymer derivatives with desirable properties. Soy flour adhesives were reported to be produced from insoluble soy flour via sodium periodate oxidation. Not only starch but also other sources like protein, tannin, and lignin can be used as raw materials to generate valuable products [63, 72, 73].
4.1.2 Reduction depolymerization
The reductive depolymerization of lignin is a method that harnesses hydrogen’s potential by selectively snipping its C∙O and C∙C bonds through a medley of hydrogenation, hydrogenolysis, and hydrodeoxygenation reactions. Biofuel containing high oxygen can cause chemical instability and high viscosity, which were found to be disadvantages for industrial applications. Subsequently, the reductive depolymerization can generate a high H/C ratio or hydrogen-to-oxygen ratio of lignin monomer products by eliminating the excess oxygen into the water via hydrodeoxygenation, this process can be beneficial for biofuel or biochemical productions. Various catalysts are used as reduction depolymerization agents, including ruthenium (Ru), palladium (Pd), gold (Au), and nickel (Ni), and the combination with carbon (C) or carbon nanotube (CNT).
Employing CNT as a support for Ru catalysts and positioning these catalysts at the interface of water and oil droplets to facilitate hydrodeoxygenation of vanillin to
4.2 Thermal depolymerization
Exploiting the complex chemistry of lignin, a promising avenue for sustainable resource utilization lies in thermal depolymerization. This process, often akin to pyrolysis, utilizes controlled heat in the absence of oxygen to break down the intricate lignin network. Researchers can guide depolymerization toward desired outcomes by carefully manipulating temperature, pressure, and reaction time. At lower temperatures, selective cleavage of specific bonds yields valuable phenolic monomers and oligomers, paving the way for green chemicals and bioplastics. Conversely, higher temperatures promote extensive fragmentation, generating bio-oils with potential applications in fuels and lubricants. Understanding the complex interplay between reaction conditions and product distribution remains a key challenge. By employing advanced characterization techniques and computational modeling, researchers continuously refine thermal depolymerization protocols, aiming to unlock the full potential of this transformative technology for a sustainable future.
4.2.1 Pyrolysis and microwave
Pyrolysis offers a promising approach to unlocking the hidden energy potential within LB and residual wastes like lignin. The method involves high temperatures ranging from 250 to 900°C and an inert atmosphere in the lack or limited level of oxygen as an oxidizing factor and transforms the biomass into vapor form of several organic compounds, such as alcohols, benzene, and phenols that afterward processes into valuable products like bio-oil by condensation, bio-char (bio-charcoal) as a solid residue, and leftover gases into syngas [77].
Temperature and duration reign in shaping the products of pyrolysis reactions. During slow pyrolysis, low temperatures and extended holding times favor ample syngas production that can be used as cooking gas and charcoal formation, ideal for applications like biofertilizers or adsorbents. Conversely, fast pyrolysis, with its high temperatures (800°C up to 900°C) and fleeting holding times (less than 2 s), boosts bio-oil yields, enriched with aromatic compounds from lignin breakdown [78]. However, optimizing this fast pyrolysis requires careful tuning for temperature, holding time, and flow rate, all of which influence the product distribution, highlighting the intricate interplay between these variables. Aside from temperature and time, the feedstock type also plays an important role in product distribution.
Microwave implementation has gained popularity for pyrolysis due to its rapid heating rates and uniform heating at the molecular level in comparison with conventional pyrolysis. Pyrolyzing sewage sludge at 490°C with 400 W of microwave power produced a considerably higher bio-oil content (49.7 wt%) compared to conventional heating at 500°C (36.7 wt%) [79, 80]. The fast-holding time of oil in the microwave avoids secondary cracking to gaseous products, resulting in a higher bio-oil yield [81]. Despite the advantages of microwave-assisted pyrolysis, challenges remain in its practical application, particularly for lignin depolymerization. The product selectivity in pyrolysis tends to be low, and separating the various products poses a significant challenge. This limitation in product separation could hinder the widespread adoption of microwave-assisted pyrolysis for lignin depolymerization. Researchers are addressing these challenges to improve the efficiency and feasibility of this innovative pyrolytic approach.
4.2.2 Gasification
Gasification is an alternative thermal depolymerization of LB into synthetic fuel, such as methanol, dimethyl ether, and paraffin, through the Fischer-Tropsch mechanism [82]. The process is conducted at extremely high temperatures above 800°C while maintaining the presence of air or oxygen as an oxidizing agent [83]. The sequence of the gasification process includes oxidation by combustion that forms CO and CO2 (from char) and water (from H2 of syngas), drying steps to eliminate the moisture in LB, then pyrolysis decomposition, and lastly, reduction steps that produce CO and H2 (boudouard reaction) also CH4 and H2 (methanation reaction). The production of CO and H2 leads to the Fischer-Tropsch process [84]. Decrement of the carbon-to-hydrogen ratio through gasification enhances the product’s calorific value and simultaneously generates hydrogen, which is important for the reductive depolymerization of lignin into valuable aromatics [85].
4.3 Photocatalytic depolymerization
Photocatalytic depolymerization uses light and a photocatalyst to break down lignin into smaller molecules. The photocatalyst absorbs light, creating excited electrons and holes that initiate chemical reactions. These reactions can cleave lignin bonds, leading to the formation of various products like monomers, oligomers, and aromatic chemicals (Figure 6) [86].
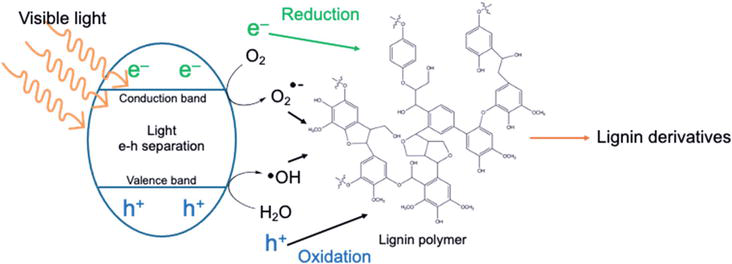
Figure 6.
In the heterogeneous photocatalytic conversion of lignin, the black arrow is an oxidation-related process, while the green arrows represent reduction-related steps within the mechanism.
Compared to traditional methods like thermochemical or biological depolymerization, photocatalysis offers several advantages, such as mild reaction conditions, lignin can be depolymerized at lower temperatures and pressures, minimizing energy consumption and potential degradation of valuable products. Second, the choice of photocatalyst and reaction conditions can potentially target-specific lignin bonds, leading to the desired products. Lastly, the sustainability aspect. Photocatalysis uses light that mostly comes from unlimited resources like sunlight and readily available resources, making it a potentially environmentally friendly approach [87]. Despite its environmental merits, some challenges arise, including low product yields, due to current methods still struggling with efficiently converting lignin into valuable products. The second is the catalyst stability, and photocatalysts can deactivate or degrade over time, requiring design improvements. The last one is reactor design for optimizing light utilization and mass transfer within the reactor, which is crucial for efficient depolymerization and scaling up for industrial implementations that require cost-effective materials and processes [88].
4.4 Electrochemical depolymerization
Electrochemical depolymerization has gained significant interest as a method to enhance the conversion of lignin from LB. This process is advantageous because it can be conducted at room temperature and in a normal atmosphere [89, 90]. The electrochemical depolymerization of lignin offers many notable advantages compared to thermochemical and chemocatalytic methods of lignin degradation. Firstly, the process does not require specific amounts of oxidants, increasing efficiency and reducing environmental impact. Secondly, environmentally friendly byproducts are produced, with water being a major part of the process [91]. Thirdly, gentle reaction conditions without requiring delicate chemicals are used [92]. Fourthly, the lignin degradation process can be precisely controlled by adjusting the electrode potential or current intensity, resulting in high selectivity for desired outcomes [93]. Additionally, combining electrochemical lignin upgrade with renewable energy harvesting is a promising approach to achieving carbon neutrality [94, 95, 96]. Electrochemical lignin depolymerization aims to selectively disassemble lignin into specific chemical intermediates or monomers. By employing particular conditions and catalysts, it is feasible to produce useful molecules such as phenols, vanillin, syringaldehyde, and other aromatic substances selectively.
The primary strategies used for lignin depolymerization based on electrocatalytic oxidation consist of four modes, i.e., mediated electrooxidation, electroenzymatic oxidation, photoelectrochemical oxidation, and direct electrooxidation (Figure 4).
In mediated electrooxidation, a redox-active mediator facilitates electron transfer between the anode and lignin polymers (as depicted by type I in Figure 7). The redox-active mediator undergoes electrochemical transformation at the anode, which is oxidized. Subsequently, the oxidized mediator reacts chemically with the lignin polymer present in the electrolyte, leading to the breakdown of the lignin polymer and the formation of its oxidized products. Following this reaction, the mediator returns to its reduced state, completing the cycle. One of the advantages of using soluble catalysts in this mediated electrooxidation process is that the lignin substrate can be present in the solution as a slurry [97]. This allows for efficient lignin processing under suitable conditions, enhancing the overall efficiency of the electrochemical oxidation process.
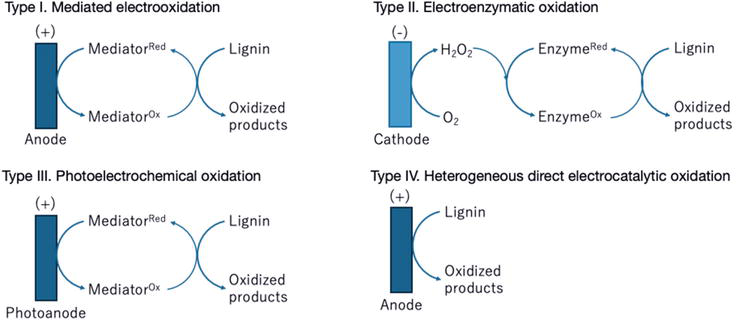
Figure 7.
Classification of electrocatalytic lignin depolymerization based on oxidation.
Under electroenzymatic oxidation conditions, a lignin depolymerization enzyme such as LiP and MnP in the solution is activated by an electrochemically generated oxidant, often H2O2. Subsequently, the activated enzyme interacts with lignin to produce oxidized lignin products (as depicted by type II in Figure 7) [98]. In this mode, lignin oxidation typically undergoes a cathodic process. Researchers have developed LiP and MnP-inspired Mn-Schiff complexes as mediators for the electroenzymatic oxidation of lignin [90]. These complexes play a role similar to redox-active mediators in mediated electrooxidation, facilitating electron transfer between the enzyme and lignin substrates, thus promoting the depolymerization of lignin.
In photoelectrochemical systems for lignin oxidation, the photogenerated hole within a photoanode is utilized to oxidize the redox mediator, thereby initiating the lignin oxidation reaction (as illustrated by type III in Figure 7) [99]. A notable example of such a system is reported by Li et al. [100], where they introduced a photoelectrochemical PINO-catalyzed lignin oxidation method. In this method, a ruthenium polypyridyl photocatalyst (RuC) is immobilized on the surface of a TiO2 photoanode. Under visible light irradiation, the excited state photocatalyst (RuC*) donates one electron to the TiO2 semiconductor, generating an oxidized state photocatalyst (RuC+). The NHPI/2,6-lutidine co-catalyst is then sufficiently oxidized to PINO radical by RuC+ in the electrolyte, initiating the lignin oxidation reaction.
In heterogeneous direct electrocatalytic oxidation mode, a solid-state catalyst serves as an electrode or is immobilized on the electrode surface, enabling direct electrochemical oxidation of the lignin polymer on the electrode surface (as depicted by type IV in Figure 7). Metal oxides have shown significant electrocatalytic activity for the oxidation of organic compounds [101, 102, 103, 104, 105, 106, 107]. Numerous studies have achieved successful heterogeneous electrocatalytic oxidation of lignin using transition metals, metal oxides, or alloys as the anode material. While theoretically highly efficient, several issues limit the effectiveness of lignin depolymerization via this method. One major issue is anode deactivation, primarily due to the formation of a passivation layer on the anode surface during lignin electrolysis, hindering active site accessibility and increasing internal resistance [102, 108, 109].
Electrochemical lignin depolymerization has obstacles concerning reaction selectivity, energy efficiency, and cost-effectiveness, notwithstanding its potential. Scientists are continuously improving the method and creating effective catalysts to enhance its feasibility for large-scale utilization of lignin.
5. Valorization of lignin by biological platforms
The past decade has witnessed a surge in microbial production of biofuels and valuable products like lipids, vanillin, biopolymers, and other valuable lignin monomeric aromatic compounds (aldehydes, acids, and alcohols) directly from lignin and its derivatives. This exciting development hinges on the efficient breakdown of lignin, the initial step in biofuel and biomaterial synthesis. Enzymatic degradation by microbes, particularly fungi, bacteria, and yeasts, offers a promising eco-friendly approach to unlocking lignin’s potential. Remarkably, these microbes produce a diverse array of lignin-degrading enzymes, including laccases, peroxidases, and dye-decolorizing peroxidases, paving the way for further advancements in lignin utilization [110]. Recent advancements in screening, strain development, and enzyme utilization strategies have further amplified their effectiveness, paving the way for a new era of efficient lignin valorization. As research delves deeper into the lignin valorization by microbial platforms, the possibilities for sustainable biofuel and biomaterial production from this once recalcitrant resource become increasingly limitless.
5.1 Lignin valorization by fungi
As nature decomposers, white-rot fungi (mainly from Basidiomycota) hold immense potential beyond their well-known ability to break down lignin [111]. White-rot fungi outperformed brown-rot fungi and soft-rot fungi in terms of capability to degrade lignin. Their versatility and efficiency make them valuable tools for a range of applications, from environmental cleanup to biofuel production, which lies in their ability to produce lignin-degrading enzymes, including laccases, lignin peroxidases (LiP), manganese peroxidases (MnP), and versatile peroxidases (VP) (Figure 8) [112]. Lignin degradation through laccases occurs through either oxidation or the acceptance of an oxygen molecule as an electron donor. LiP exhibits similarities with other peroxidases regarding their catalytic and oxidative [5]. The peroxidase enzymes can catalyze lignin encompassing phenolic and non-phenolic varieties, using H2O2. This catalytic activity is attributed to the presence of an iron protoporphyrin IX for each protein molecule in these enzymes. Specifically, LiP typically employs long-range electron transfer mechanisms to oxidize lignin compounds. In contrast, MnP utilizes Mn (III) oxidation as a diffusible intermediate for its catalytic process. Versatile peroxidases (VP), on the other hand, operate through a combination of both electron transfer and diffusible intermediate in their mode of action [113].
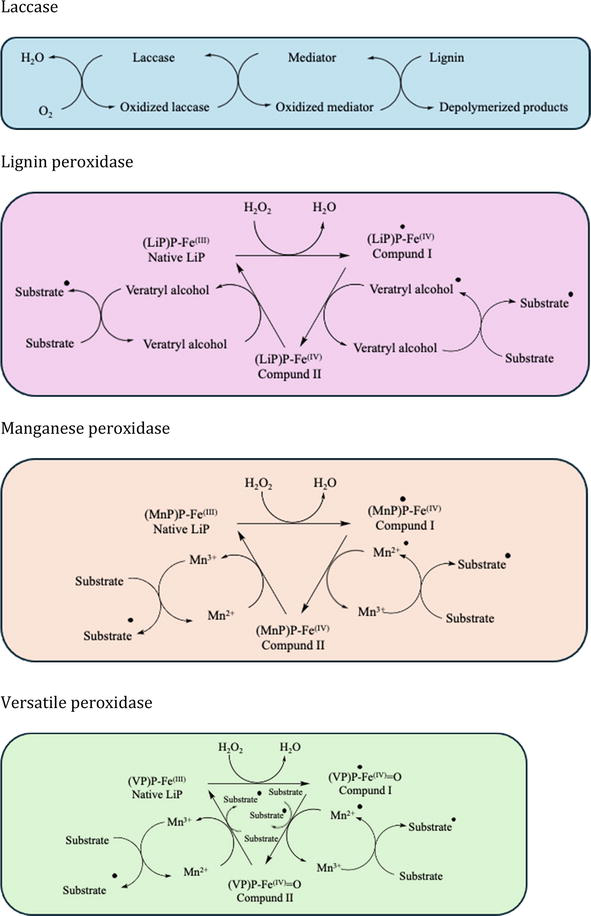
Figure 8.
Catalytic mechanism of enzymatic depolymerization.
In vitro applications leverage these enzymes to depolymerize lignin and its derivatives, presenting a promising alternative to directed-cell lignin conversion. The adoption of in vitro enzymatic conversion offers several advantages, such as enhancing substrate and enzyme interaction. This approach also holds the potential to reduce cultivation time and address ATP/NAD(P)H imbalance, showcasing its versatility and efficiency in lignin transformation processes [114].
Among other white-rot fungi,
Even though some species of white-rot fungi do not have the ability to degrade cellulose, only lignin and hemicellulose, such as
In conclusion, white-rot fungi are not just lignin-munching machines; they are versatile biocatalysts with the potential to revolutionize various industries. From environmental cleanup to biofuel production, these remarkable organisms offer a sustainable and efficient solution for a range of challenges. As we continue to unlock their potential, the future looks promising for white-rot fungi to become the impactful agents of a greener and cleaner world.
5.2 Lignin valorization by bacteria
While bacterial lignin degradation efficiency lags behind fungi, their versatility for adaptation in diverse environments is beneficial.
While aerobic bacteria have some merits for lignin utilization, anaerobic bacteria have the ability to convert lignin into carbon dioxide and methane [124, 125]. Bacteria prefer lignin with more methoxyl groups, using them as a docking point for their enzymatic attack. The process unfolds in three acts: methoxyl removal, aromatic ring cleavage, and methane formation. Methanogenic microbes turn the lignin-derived aromatics into the final act of methane production. The paper and pulp industry even hosts its anaerobic decomposers like
From the sun-drenched surface to the oxygen-deprived depths, these remarkable bacteria reveal a hidden world of lignin degradation, with each act in their play offering valuable insights for biofuel production, environmental remediation, and sustainable resource utilization.
5.3 Lignin valorization by yeast
As a part of microorganisms like fungi and bacteria, the potential of yeasts for biofuel production from lignin monomeric compounds is promising. Olegianous yeast like
While lipid production from lignin holds significant promise, exploring other valuable products derived from yeast metabolism unlocks the full potential of lignin valorization. For instance,
6. Conclusion
Lignin valorization presents a critical challenge and exciting opportunity for the biorefinery industry. The future of the biorefinery landscape hinges on effectively valorizing lignin. This intricate biopolymer offers a treasure trove of possibilities, from replacing petroleum-derived chemicals to creating novel biomaterials. Unlocking its full potential requires addressing challenges like optimizing extraction and conversion processes while ensuring economic viability. We can transform lignin from a mere byproduct into a driving force for a greener and more sustainable future through a concerted effort involving researchers, industry leaders, and policymakers.
Acknowledgments
This work was partially supported by the New Energy and Industrial Technology Development Organization (NEDO) Feasibility Study Program, Japan, the International Joint Program, Science and Technology Research Partnership for Sustainable Development (SATREPS) from the Japan Science and Technology Agency, and the Japan International Cooperation Agency (JST and JICA).
Authorship contribution statement
Conceptualization, F.J.N.P., and P.K.; data curation, F.J.N.P.; visualization, F.J.N.P. and P.K.; writing—original draft, F.J.N.P. and P.K.; supervision, P.K., C.O., and A.K. All authors have read and agreed to the published version of the manuscript.
References
- 1.
Holechek JL, Geli HME, Sawalhah MN, Valdez R. A global assessment: Can renewable energy replace fossil fuels by 2050? Sustainability. 2022; 14 :4792. DOI: 10.3390/su14084792 - 2.
York R. Do alternative energy sources displace fossil fuels? Nature Climate Change. 2012; 2 :441-443. DOI: 10.1038/nclimate1451 - 3.
Singh N, Singhania RR, Nigam PS, Dong C-D, Patel AK, Puri M. Global status of lignocellulosic biorefinery: Challenges and perspectives. Bioresource Technology. 2022; 344 :126415. DOI: 10.1016/j.biortech.2021.126415 - 4.
Matsushita Y. Conversion of technical lignins to functional materials with retained polymeric properties. Journal of Wood Science. 2015; 61 :230-250. DOI: 10.1007/s10086-015-1470-2 - 5.
Putra FJN, Kahar P, Kondo A, Ogino C. Valorization of lignin and its derivatives using yeast. PRO. 2022; 10 :2004. DOI: 10.3390/pr10102004 - 6.
Wang H, Pu Y, Ragauskas A, Yang B. From lignin to valuable products–strategies, challenges, and prospects. Bioresource Technology. 2019; 271 :449-461. DOI: 10.1016/j.biortech.2018.09.072 - 7.
Zhang C. Lignocellulosic Ethanol: Technology and Economics. Alcohol Fuels - Current Technologies and Future Prospect. London, UK: IntechOpen; 2020. DOI: 10.5772/intechopen.86701 - 8.
Mankar AR, Modak A, Pant KK. Recent advances in the valorization of lignin: A key focus on pretreatment, characterization, and catalytic depolymerization strategies for future biorefineries. Advanced Sustainable Systems. 2022; 6 :2100299. DOI: 10.1002/adsu.202100299 - 9.
Kenny JK, Medlin JW, Beckham GT. Quantification of phenolic hydroxyl groups in lignin via 19 F NMR spectroscopy. ACS Sustainable Chemistry & Engineering. 2023; 11 :5644-5655. DOI: 10.1021/acssuschemeng.3c00115 - 10.
Shakeel U, Li X, Wang B, Geng F, Rehman MSU, Zhang K, et al. Structural characterizations of lignins extracted under same severity using different acids. International Journal of Biological Macromolecules. 2022; 194 :204-212. DOI: 10.1016/j.ijbiomac.2021.11.171 - 11.
Ponomarenko J, Lauberts M, Dizhbite T, Lauberte L, Jurkjane V, Telysheva G. Antioxidant activity of various lignins and lignin-related phenylpropanoid units with high and low molecular weight. Holzforschung. 2015; 69 :795-805. DOI: 10.1515/hf-2014-0280 - 12.
Sun Z, Fridrich B, de Santi A, Elangovan S, Barta K. Bright side of lignin depolymerization: Toward new platform chemicals. Chemical Reviews. 2018; 118 :614-678. DOI: 10.1021/acs.chemrev.7b00588 - 13.
Upton BM, Kasko AM. Strategies for the conversion of lignin to high-value polymeric materials: Review and perspective. Chemical Reviews. 2016; 116 :2275-2306. DOI: 10.1021/acs.chemrev.5b00345 - 14.
Li C, Zhao X, Wang A, Huber GW, Zhang T. Catalytic transformation of lignin for the production of chemicals and fuels. Chemical Reviews. 2015; 115 :11559-11624. DOI: 10.1021/acs.chemrev.5b00155 - 15.
Pu Y, Cao S, Ragauskas AJ. Application of quantitative 31P NMR in biomass lignin and biofuel precursors characterization. Energy & Environmental Science. 2011; 4 :3154. DOI: 10.1039/c1ee01201k - 16.
Houfani AA, Anders N, Spiess AC, Baldrian P, Benallaoua S. Insights from enzymatic degradation of cellulose and hemicellulose to fermentable sugars– A review. Biomass and Bioenergy. 2020; 134 :105481. DOI: 10.1016/j.biombioe.2020.105481 - 17.
Yuan Y, Jiang B, Chen H, Wu W, Wu S, Jin Y, et al. Recent advances in understanding the effects of lignin structural characteristics on enzymatic hydrolysis. Biotechnology for Biofuels. 2021; 14 :205. DOI: 10.1186/s13068-021-02054-1 - 18.
Lourenço A, Pereira H. Compositional Variability of Lignin in Biomass. Lignin - Trends and Applications. London, UK: InTech; 2018. DOI: 10.5772/intechopen.71208 - 19.
Anderson EM, Stone ML, Katahira R, Reed M, Muchero W, Ramirez KJ, et al. Differences in S/G ratio in natural poplar variants do not predict catalytic depolymerization monomer yields. Nature Communications. 2019; 10 :2033. DOI: 10.1038/s41467-019-09986-1 - 20.
Yoo CG, Dumitrache A, Muchero W, Natzke J, Akinosho H, Li M, et al. Significance of lignin S/G ratio in biomass recalcitrance of Populus trichocarpa variants for bioethanol production. ACS Sustainable Chemistry & Engineering. 2018;6 :2162-2168. DOI: 10.1021/acssuschemeng.7b03586 - 21.
Meng X, Parikh A, Seemala B, Kumar R, Pu Y, Wyman CE, et al. Characterization of fractional cuts of co-solvent enhanced lignocellulosic fractionation lignin isolated by sequential precipitation. Bioresource Technology. 2019; 272 :202-208. DOI: 10.1016/j.biortech.2018.09.130 - 22.
Shi Z, Xu G, Deng J, Dong M, Murugadoss V, Liu C, et al. Structural characterization of lignin from D. Sinicus by FTIR and NMR techniques. Green Chemistry Letters and Reviews. 2019;12 :235-243. DOI: 10.1080/17518253.2019.1627428 - 23.
Alves CT, Peters MA, Onwudili JA. Application of thermogravimetric analysis method for the characterisation of products from triglycerides during biodiesel production. Journal of Analytical and Applied Pyrolysis. 2022; 168 :105766. DOI: 10.1016/j.jaap.2022.105766 - 24.
Eugenio ME, Martín-Sampedro R, Santos JI, Wicklein B, Ibarra D. Chemical, thermal and antioxidant properties of Lignins solubilized during soda/AQ pulping of Orange and olive tree pruning residues. Molecules. 2021; 26 :3819. DOI: 10.3390/molecules26133819 - 25.
Meng X, Crestini C, Ben H, Hao N, Pu Y, Ragauskas AJ, et al. Determination of hydroxyl groups in biorefinery resources via quantitative 31P NMR spectroscopy. Nature Protocols. 2019; 14 :2627-2647. DOI: 10.1038/s41596-019-0191-1 - 26.
Gioia C, Lo Re G, Lawoko M, Berglund L. Tunable thermosetting epoxies based on fractionated and well-characterized Lignins. Journal of the American Chemical Society. 2018; 140 :4054-4061. DOI: 10.1021/jacs.7b13620 - 27.
Gallego-García M, Moreno AD, Manzanares P, Negro MJ, Duque A. Recent advances on physical technologies for the pretreatment of food waste and lignocellulosic residues. Bioresource Technology. 2023; 369 :128397. DOI: 10.1016/j.biortech.2022.128397 - 28.
Shah AA, Seehar TH, Sharma K, Toor SS. Biomass pretreatment technologies. In: Hydrocarbon Biorefinery. Amsterdam, Netherlands: Elsevier; 2022. pp. 203-228. DOI: 10.1016/B978-0-12-823306-1.00014-5 - 29.
Arce C, Kratky L. Mechanical pretreatment of lignocellulosic biomass toward enzymatic/fermentative valorization. IScience. 2022; 25 :104610. DOI: 10.1016/j.isci.2022.104610 - 30.
Jablonowski ND, Pauly M, Dama M. Microwave assisted pretreatment of Szarvasi (Agropyron elongatum) biomass to enhance enzymatic saccharification and direct glucose production. Frontiers in Plant Science. 2022; 12 :767254. DOI: 10.3389/fpls.2021.767254 - 31.
Gatt E, Rigal L, Vandenbossche V. Biomass pretreatment with reactive extrusion using enzymes: A review. Industrial Crops and Products. 2018; 122 :329-339. DOI: 10.1016/j.indcrop.2018.05.069 - 32.
Ashokkumar M. The characterization of acoustic cavitation bubbles – An overview. Ultrasonics Sonochemistry. 2011; 18 :864-872. DOI: 10.1016/j.ultsonch.2010.11.016 - 33.
Flores EMM, Cravotto G, Bizzi CA, Santos D, Iop GD. Ultrasound-assisted biomass valorization to industrial interesting products: State-of-the-art, perspectives and challenges. Ultrasonics Sonochemistry. 2021; 72 :105455. DOI: 10.1016/j.ultsonch.2020.105455 - 34.
Wang W, Wang X, Zhang Y, Yu Q , Tan X, Zhuang X, et al. Effect of sodium hydroxide pretreatment on physicochemical changes and enzymatic hydrolysis of herbaceous and woody lignocelluloses. Industrial Crops and Products. 2020; 145 :112145. DOI: 10.1016/j.indcrop.2020.112145 - 35.
Singh A, Kushwaha A, Sen S, Goswami S, Katiyar S, Kumar A, et al. Recent advancement in microwave-assisted pyrolysis for biooil production. In: Waste-to-Energy Approaches Towards Zero Waste. Amsterdam, Netherlands: Elsevier; 2022. pp. 197-219. DOI: 10.1016/B978-0-323-85387-3.00014-8 - 36.
Shen J, Zhao C, Liu G, Chen C. Enhancing the performance on anaerobic digestion of vinegar residue by sodium hydroxide pretreatment. Waste and Biomass Valorization. 2017; 8 :1119-1126. DOI: 10.1007/s12649-016-9666-2 - 37.
Shetty DJ, Kshirsagar P, Tapadia-Maheshwari S, Lanjekar V, Singh SK, Dhakephalkar PK. Alkali pretreatment at ambient temperature: A promising method to enhance biomethanation of rice straw. Bioresource Technology. 2017; 226 :80-88. DOI: 10.1016/j.biortech.2016.12.003 - 38.
Sakuragi K, Igarashi K, Samejima M. Application of ammonia pretreatment to enable enzymatic hydrolysis of hardwood biomass. Polymer Degradation and Stability. 2018; 148 :19-25. DOI: 10.1016/j.polymdegradstab.2017.12.008 - 39.
Shrotri A, Kobayashi H, Fukuoka A. Catalytic Conversion of Structural Carbohydrates and Lignin to Chemicals. Advances in Catalysis. Amsterdam, Netherlands: Elsevier; 2017. pp. 59-123. DOI: 10.1016/bs.acat.2017.09.002 - 40.
Jönsson LJ, Martín C. Pretreatment of lignocellulose: Formation of inhibitory by-products and strategies for minimizing their effects. Bioresource Technology. 2016; 199 :103-112. DOI: 10.1016/j.biortech.2015.10.009 - 41.
Qing Q , Huang M, He Y, Wang L, Zhang Y. Dilute oxalic acid pretreatment for high total sugar recovery in pretreatment and subsequent enzymatic hydrolysis. Applied Biochemistry and Biotechnology. 2015; 177 :1493-1507. DOI: 10.1007/s12010-015-1829-2 - 42.
Edwiges T, Roda-Serrat MC, Segovia-Hernández JG, Sánchez-Ramírez E, Tronci S, Errico M. Sustainable bioalcohol production: Pretreatment, separation, and control strategies leading to sustainable processes. In: Biofuels and Biorefining. Amsterdam, Netherlands: Elsevier; 2022. pp. 41-85. DOI: 10.1016/B978-0-12-824117-2.00004-1 - 43.
Li J, Shi S, Tu M, Via B, Sun FF, Adhikari S. Detoxification of Organosolv-pretreated pine prehydrolysates with anion resin and cysteine for butanol fermentation. Applied Biochemistry and Biotechnology. 2018; 186 :662-680. DOI: 10.1007/s12010-018-2769-4 - 44.
Bhatia SK, Kim J, Song H-S, Kim HJ, Jeon J-M, Sathiyanarayanan G, et al. Microbial biodiesel production from oil palm biomass hydrolysate using marine Rhodococcus sp. YHY01. Bioresource Technology. 2017; 233 :99-109. DOI: 10.1016/j.biortech.2017.02.061 - 45.
Niju S, Swathika M, Balajii M. Pretreatment of lignocellulosic sugarcane leaves and tops for bioethanol production. In: Lignocellulosic Biomass to Liquid Biofuels. Amsterdam, Netherlands: Elsevier; 2020. pp. 301-324. DOI: 10.1016/B978-0-12-815936-1.00010-1 - 46.
Liu C-G, Li K, Wen Y, Geng B-Y, Liu Q , Lin Y-H. Bioethanol: New Opportunities for an Ancient Product. Advances in Bioenergy. Amsterdam, Netherlands: Elsevier; 2019. pp. 1-34. DOI: 10.1016/bs.aibe.2018.12.002 - 47.
Yoo CG, Pu Y, Ragauskas AJ. Ionic liquids: Promising green solvents for lignocellulosic biomass utilization. Current Opinion in Green and Sustainable Chemistry. 2017; 5 :5-11. DOI: 10.1016/j.cogsc.2017.03.003 - 48.
Xu J, Liu B, Hou H, Hu J. Pretreatment of eucalyptus with recycled ionic liquids for low-cost biorefinery. Bioresource Technology. 2017; 234 :406-414. DOI: 10.1016/j.biortech.2017.03.081 - 49.
Raj JJ, Magaret S, Pranesh M, Lethesh KC, Devi WC, Mutalib MIA. Dual functionalized imidazolium ionic liquids as a green solvent for extractive desulfurization of fuel oil: Toxicology and mechanistic studies. Journal of Cleaner Production. 2019; 213 :989-998. DOI: 10.1016/j.jclepro.2018.12.207 - 50.
Usmani Z, Sharma M, Gupta P, Karpichev Y, Gathergood N, Bhat R, et al. Ionic liquid based pretreatment of lignocellulosic biomass for enhanced bioconversion. Bioresource Technology. 2020; 304 :123003. DOI: 10.1016/j.biortech.2020.123003 - 51.
Binder JB, Gray MJ, White JF, Zhang ZC, Holladay JE. Reactions of lignin model compounds in ionic liquids. Biomass and Bioenergy. 2009; 33 :1122-1130. DOI: 10.1016/j.biombioe.2009.03.006 - 52.
Yang Y, Fan H, Song J, Meng Q , Zhou H, Wu L, et al. Free radical reaction promoted by ionic liquid: A route for metal-free oxidation depolymerization of lignin model compound and lignin. Chemical Communications. 2015; 51 :4028-4031. DOI: 10.1039/C4CC10394G - 53.
Arif, Hakimi Saadon SZ, Osman NB, Yusup S. Pretreatment of fiber-based biomass material for lignin extraction. In: Value-Chain of Biofuels. Amsterdam, Netherlands: Elsevier; 2022. pp. 105-135. DOI: 10.1016/B978-0-12-824388-6.00024-5 - 54.
Chu Q , Tong W, Chen J, Wu S, Jin Y, Hu J, et al. Organosolv pretreatment assisted by carbocation scavenger to mitigate surface barrier effect of lignin for improving biomass saccharification and utilization. Biotechnology for Biofuels. 2021; 14 :136. DOI: 10.1186/s13068-021-01988-w - 55.
Yang L, Zheng T, Huang C, Yao J. Using deep eutectic solvent pretreatment for enhanced enzymatic saccharification and lignin utilization of masson pine. Renewable Energy. 2022; 195 :681-687. DOI: 10.1016/j.renene.2022.06.066 - 56.
Zdanowicz M, Wilpiszewska K, Spychaj T. Deep eutectic solvents for polysaccharides processing. A review. Carbohydrate Polymers. 2018; 200 :361-380. DOI: 10.1016/j.carbpol.2018.07.078 - 57.
Modak A, Mankar AR, Pant KK, Bhaumik A. Mesoporous porphyrin-silica nanocomposite as solid acid catalyst for high yield synthesis of HMF in water. Molecules. 2021; 26 :2519. DOI: 10.3390/molecules26092519 - 58.
Walsh DJ, Hyatt MG, Miller SA, Guironnet D. Recent trends in catalytic polymerizations. ACS Catalysis. 2019; 9 :11153-11188. DOI: 10.1021/acscatal.9b03226 - 59.
Tarabanko V, Tarabanko N. Catalytic oxidation of Lignins into the aromatic aldehydes: General process trends and development prospects. International Journal of Molecular Sciences. 2017; 18 :2421. DOI: 10.3390/ijms18112421 - 60.
Liu C, Wu S, Zhang H, Xiao R. Catalytic oxidation of lignin to valuable biomass-based platform chemicals: A review. Fuel Processing Technology. 2019; 191 :181-201. DOI: 10.1016/j.fuproc.2019.04.007 - 61.
Dai J, Patti AF, Saito K. Recent developments in chemical degradation of lignin: Catalytic oxidation and ionic liquids. Tetrahedron Letters. 2016; 57 :4945-4951. DOI: 10.1016/j.tetlet.2016.09.084 - 62.
Ouyang X, Tan Y, Qiu X. Oxidative degradation of lignin for producing monophenolic compounds. Journal of Fuel Chemistry and Technology. 2014; 42 :677-682. DOI: 10.1016/S1872-5813(14)60030-X - 63.
Chen X, Xi X, Pizzi A, Fredon E, Du G, Gerardin C, et al. Oxidized demethylated lignin as a bio-based adhesive for wood bonding. The Journal of Adhesion. 2021; 97 :873-890. DOI: 10.1080/00218464.2019.1710830 - 64.
Mathieu Y, Vidal JD, Arribas Martínez L, Abad Fernández N, Iborra S, Corma A. Molecular oxygen lignin depolymerization: An insight into the stability of phenolic monomers. ChemSusChem. 2020; 13 :4743-4758. DOI: 10.1002/cssc.202001295 - 65.
Kumar A, Biswas B, Saini K, Kumar A, Kumar J, Krishna BB, et al. Effect of hydrogen peroxide on the depolymerization of prot lignin. Industrial Crops and Products. 2020; 150 :112355. DOI: 10.1016/j.indcrop.2020.112355 - 66.
Gierer J. Chemistry of delignification. Wood Science and Technology. 1986; 20 :1-33. DOI: 10.1007/BF00350692 - 67.
Cymbaluk NF, Neudoerffer TS. A quantitative gas-liquid chromatographic determination of aromatic aldehydes and acids from nitrobenzene oxidation of lignin. Journal of Chromatography. A. 1970; 51 :167-174. DOI: 10.1016/S0021-9673(01)96851-7 - 68.
Deng H, Zhang H, Wu Z, Tian X, Jiang X, Guo W. Rules and mechanism for the oxidation of lignin-based aromatic aldehyde under alkaline wet oxygen. BioResources. 2020; 15 :3487-3503. DOI: 10.15376/biores.15.2.3487-3503 - 69.
Tang P-L, Hassan O, Maskat MY, Badri K. Production of monomeric aromatic compounds from oil palm empty fruit bunch Fiber lignin by chemical and enzymatic methods. BioMed Research International. 2015; 2015 :1-14. DOI: 10.1155/2015/891539 - 70.
Deng H, Lin L, Liu S. Catalysis of Cu-doped Co-based perovskite-type oxide in wet oxidation of lignin to produce aromatic aldehydes. Energy & Fuels. 2010; 24 :4797-4802. DOI: 10.1021/ef100768e - 71.
Voitl T, Rudolf von Rohr P. Oxidation of lignin using aqueous polyoxometalates in the presence of alcohols. ChemSusChem. 2008; 1 :763-769. DOI: 10.1002/cssc.200800050 - 72.
Xi X, Pizzi A, Frihart CR, Lorenz L, Gerardin C. Tannin plywood bioadhesives with non-volatile aldehydes generation by specific oxidation of mono- and disaccharides. International Journal of Adhesion and Adhesives. 2020; 98 :102499. DOI: 10.1016/j.ijadhadh.2019.102499 - 73.
Frihart CR, Pizzi A, Xi X, Lorenz LF. Reactions of soy flour and soy protein by non-volatile aldehydes generation by specific oxidation. Polymers (Basel). 2019; 11 :1478. DOI: 10.3390/polym11091478 - 74.
Yang X, Liang Y, Zhao X, Song Y, Hu L, Wang X, et al. Au/CNTs catalyst for highly selective hydrodeoxygenation of vanillin at the water/oil interface. RSC Advances. 2014; 4 :31932-31936. DOI: 10.1039/C4RA04692G - 75.
Sunil More G, Rajendra Kanchan D, Banerjee A, Srivastava R. Selective catalytic hydrodeoxygenation of vanillin to 2-Methoxy-4-methyl phenol and 4-methyl cyclohexanol over Pd/CuFe2O4 and PdNi/CuFe2O4 catalysts. Chemical Engineering Journal. 2023; 462 :142110. DOI: 10.1016/j.cej.2023.142110 - 76.
Zhang J, Teo J, Chen X, Asakura H, Tanaka T, Teramura K, et al. A series of NiM (M = Ru, Rh, and Pd) bimetallic catalysts for effective lignin hydrogenolysis in water. ACS Catalysis. 2014; 4 :1574-1583. DOI: 10.1021/cs401199f - 77.
Zevallos Torres LA, Lorenci Woiciechowski A, de Andrade Tanobe VO, Karp SG, Guimarães Lorenci LC, Faulds C, et al. Lignin as a potential source of high-added value compounds: A review. Journal of Cleaner Production. 2020; 263 :121499. DOI: 10.1016/j.jclepro.2020.121499 - 78.
Beneroso D, Monti T, Kostas ET, Robinson J. Microwave pyrolysis of biomass for bio-oil production: Scalable processing concepts. Chemical Engineering Journal. 2017; 316 :481-498. DOI: 10.1016/j.cej.2017.01.130 - 79.
Tian Y, Zuo W, Ren Z, Chen D. Estimation of a novel method to produce bio-oil from sewage sludge by microwave pyrolysis with the consideration of efficiency and safety. Bioresource Technology. 2011; 102 :2053-2061. DOI: 10.1016/j.biortech.2010.09.082 - 80.
Xie Q , Chen Z, Zhou Y, Pan T, Duan Y, Yu S, et al. Efficient treatment of oily sludge via fast microwave-assisted pyrolysis, followed by thermal plasma vitrification. Molecules. 2023; 28 :4036. DOI: 10.3390/molecules28104036 - 81.
Halim SA, Swithenbank J. Simulation study of parameters influencing microwave heating of biomass. Journal of the Energy Institute. 2019; 92 :1191-1212. DOI: 10.1016/j.joei.2018.05.010 - 82.
Sikarwar VS, Zhao M, Fennell PS, Shah N, Anthony EJ. Progress in biofuel production from gasification. Progress in Energy and Combustion Science. 2017; 61 :189-248. DOI: 10.1016/j.pecs.2017.04.001 - 83.
Yang X, Igalavithana AD, Oh S-E, Nam H, Zhang M, Wang C-H, et al. Characterization of bioenergy biochar and its utilization for metal/metalloid immobilization in contaminated soil. Science of the Total Environment. 2018; 640-641 :704-713. DOI: 10.1016/j.scitotenv.2018.05.298 - 84.
Molino A, Chianese S, Musmarra D. Biomass gasification technology: The state of the art overview. Journal of Energy Chemistry. 2016; 25 :10-25. DOI: 10.1016/j.jechem.2015.11.005 - 85.
Cao Y, Chen SS, Zhang S, Ok YS, Matsagar BM, Wu KC-W, et al. Advances in lignin valorization towards bio-based chemicals and fuels: Lignin biorefinery. Bioresource Technology. 2019; 291 :121878. DOI: 10.1016/j.biortech.2019.121878 - 86.
Xiang Z, Han W, Deng J, Zhu W, Zhang Y, Wang H. Photocatalytic conversion of lignin into chemicals and fuels. ChemSusChem. 2020; 13 :4199-4213. DOI: 10.1002/cssc.202000601 - 87.
Parkatzidis K, Truong NP, Matyjaszewski K, Anastasaki A. Photocatalytic ATRP depolymerization: Temporal control at low ppm of catalyst concentration. Journal of the American Chemical Society. 2023; 145 :21146-21151. DOI: 10.1021/jacs.3c05632 - 88.
Xu J, Zhou Z, Liu M, Wang J, Zhang L. Photocatalytic depolymerization of lignin via oxidizing cleavage of Cα-Cβ bonds in micellar aqueous media. International Journal of Biological Macromolecules. 2023; 245 :125476. DOI: 10.1016/j.ijbiomac.2023.125476 - 89.
Du X, Zhang H, Sullivan KP, Gogoi P, Deng Y. Electrochemical lignin conversion. ChemSusChem. 2020; 13 :4318-4343. DOI: 10.1002/cssc.202001187 - 90.
Yang C, Maldonado S, Stephenson CRJ. Electrocatalytic lignin oxidation. ACS Catalysis. 2021; 11 :10104-10114. DOI: 10.1021/acscatal.1c01767 - 91.
Grainger A, Smith G. The role of low carbon and high carbon materials in carbon neutrality science and carbon economics. Current Opinion in Environment Sustainability. 2021; 49 :164-189. DOI: 10.1016/j.cosust.2021.06.006 - 92.
May AS, Biddinger EJ. Strategies to control electrochemical hydrogenation and hydrogenolysis of furfural and minimize undesired side reactions. ACS Catalysis. 2020; 10 :3212-3221. DOI: 10.1021/acscatal.9b05531 - 93.
Wang AQ , Li CZ, Zheng MY, Zhang T. Heterogeneous catalysts for biomass conversion. In: The Role of Green Chemistry in Biomass Processing and Conversion. Hoboken: John Wiley & Sons, Inc; 2012. DOI: 10.1002/9781118449400 - 94.
Diaz LA, Lister TE, Rae C, Wood ND. Anion exchange membrane Electrolyzers as alternative for upgrading of biomass-derived molecules. ACS Sustainable Chemistry & Engineering. 2018; 6 :8458-8467. DOI: 10.1021/acssuschemeng.8b00650 - 95.
Andrews E, Lopez-Ruiz JA, Egbert JD, Koh K, Sanyal U, Song M, et al. Performance of base and Noble metals for electrocatalytic hydrogenation of bio-oil-derived oxygenated compounds. ACS Sustainable Chemistry & Engineering. 2020; 8 :4407-4418. DOI: 10.1021/acssuschemeng.9b07041 - 96.
Tu Q , Parvatker A, Garedew M, Harris C, Eckelman M, Zimmerman JB, et al. Electrocatalysis for chemical and fuel production: Investigating climate change mitigation potential and economic feasibility. Environmental Science & Technology. 2021; 55 :3240-3249. DOI: 10.1021/acs.est.0c07309 - 97.
Rafiee M, Alherech M, Karlen SD, Stahl SS. Electrochemical aminoxyl-mediated oxidation of primary alcohols in lignin to carboxylic acids: Polymer modification and depolymerization. Journal of the American Chemical Society. 2019; 141 :15266-15276. DOI: 10.1021/jacs.9b07243 - 98.
Ko M, Pham LTM, Sa YJ, Woo J, Nguyen TVT, Kim JH, et al. Unassisted solar lignin valorisation using a compartmented photo-electro-biochemical cell. Nature Communications. 2019; 10 :5123. DOI: 10.1038/s41467-019-13022-7 - 99.
Li T, Kasahara T, He J, Dettelbach KE, Sammis GM, Berlinguette CP. Photoelectrochemical oxidation of organic substrates in organic media. Nature Communications. 2017; 8 :390. DOI: 10.1038/s41467-017-00420-y - 100.
Li S, Li Z-J, Yu H, Sytu MR, Wang Y, Beeri D, et al. Solar-driven lignin oxidation via hydrogen atom transfer with a dye-sensitized TiO2 photoanode. ACS Energy Letters. 2020; 5 :777-784. DOI: 10.1021/acsenergylett.9b02391 - 101.
Bailey A, Brooks HM. Electrolytic oxidation of lignin. Journal of the American Chemical Society. 1946; 68 :445-446. DOI: 10.1021/ja01207a029 - 102.
Parpot P, Bettencourt AP, Carvalho AM, Belgsir EM. Biomass conversion: Attempted electrooxidation of lignin for vanillin production. Journal of Applied Electrochemistry. 2000; 30 :727-731. DOI: 10.1023/A:1004003613883 - 103.
Tolba R, Tian M, Wen J, Jiang Z-H, Chen A. Electrochemical oxidation of lignin at IrO2-based oxide electrodes. Journal of Electroanalytical Chemistry. 2010; 649 :9-15. DOI: 10.1016/j.jelechem.2009.12.013 - 104.
Lan C, Fan H, Shang Y, Shen D, Li G. Electrochemically catalyzed conversion of cornstalk lignin to aromatic compounds: An integrated process of anodic oxidation of a Pb/PbO2 electrode and hydrogenation of a nickel cathode in sodium hydroxide solution. Sustainable Energy & Fuels. 2020; 4 :1828-1836. DOI: 10.1039/C9SE00942F - 105.
Liu M, Wen Y, Qi J, Zhang S, Li G. Fine chemicals prepared by bamboo lignin degradation through electrocatalytic redox between Cu cathode and Pb/PbO2 anode in alkali solution. ChemistrySelect. 2017; 2 :4956-4962. DOI: 10.1002/slct.201700881 - 106.
Cai P, Fan H, Cao S, Qi J, Zhang S, Li G. Electrochemical conversion of corn Stover lignin to biomass-based chemicals between Cu/Ni Mo Co cathode and Pb/PbO2 anode in alkali solution. Electrochimica Acta. 2018; 264 :128-139. DOI: 10.1016/j.electacta.2018.01.111 - 107.
Jia Y, Wen Y, Han X, Qi J, Liu Z, Zhang S, et al. Electrocatalytic degradation of rice straw lignin in alkaline solution through oxidation on a Ti/SnO2 –Sb2O3/α-PbO2/β-PbO2 anode and reduction on an iron or tin doped titanium cathode. Catalysis Science & Technology. 2018; 8 :4665-4677. DOI: 10.1039/C8CY00307F - 108.
Ežerskis Z, Jusys Z. Electropolymerization of chlorinated phenols on a Pt electrode in alkaline solution part I: A cyclic voltammetry study. Journal of Applied Electrochemistry. 2001; 31 :1117-1124. DOI: 10.1023/A:1012280216273 - 109.
Bruno F, Pham MC, Dubois JE. Polaromicrotribometric study of polyphenylene oxide film formation on metal electrodes by electrolysis of disubstituted phenols. Electrochimica Acta. 1977; 22 :451-457. DOI: 10.1016/0013-4686(77)85100-1 - 110.
Kumar A, Chandra R. Ligninolytic enzymes and its mechanisms for degradation of lignocellulosic waste in environment. Heliyon. 2020; 6 :e03170. DOI: 10.1016/j.heliyon.2020.e03170 - 111.
Weng C, Peng X, Han Y. Depolymerization and conversion of lignin to value-added bioproducts by microbial and enzymatic catalysis. Biotechnology for Biofuels. 2021; 14 :84. DOI: 10.1186/s13068-021-01934-w - 112.
de Gonzalo G, Colpa DI, Habib MHM, Fraaije MW. Bacterial enzymes involved in lignin degradation. Journal of Biotechnology. 2016; 236 :110-119. DOI: 10.1016/j.jbiotec.2016.08.011 - 113.
Dashora K, Gattupalli M, Tripathi GD, Javed Z, Singh S, Tuohy M, et al. Fungal assisted valorisation of polymeric lignin: Mechanism, enzymes and perspectives. Catalysts. 2023; 13 :149. DOI: 10.3390/catal13010149 - 114.
Zhang Y-HP. Production of biofuels and biochemicals by in vitro synthetic biosystems: Opportunities and challenges. Biotechnology Advances. 2015; 33 :1467-1483. DOI: 10.1016/j.biotechadv.2014.10.009 - 115.
Zeng G-M, Zhao M-H, Huang D-L, Lai C, Huang C, Wei Z, et al. Purification and biochemical characterization of two extracellular peroxidases from Phanerochaete chrysosporium responsible for lignin biodegradation. International Biodeterioration & Biodegradation. 2013; 85 :166-172. DOI: 10.1016/j.ibiod.2013.07.005 - 116.
Singh D, Zeng J, Laskar DD, Deobald L, Hiscox WC, Chen S. Investigation of wheat straw biodegradation by Phanerochaete chrysosporium. Biomass and Bioenergy. 2011; 35 :1030-1040. DOI: 10.1016/j.biombioe.2010.11.021 - 117.
Koncsag CI, Eastwood D, Collis AEC, Coles SR, Clark AJ, Kirwan K, et al. Extracting valuable compounds from straw degraded by Pleurotus ostreatus. Resources, Conservation and Recycling. 2012; 59 :14-22. DOI: 10.1016/j.resconrec.2011.04.007 - 118.
Wong DWS. Structure and action mechanism of ligninolytic enzymes. Applied Biochemistry and Biotechnology. 2009; 157 :174-209. DOI: 10.1007/s12010-008-8279-z - 119.
Salvachúa D, Katahira R, Cleveland NS, Khanna P, Resch MG, Black BA, et al. Lignin depolymerization by fungal secretomes and a microbial sink. Green Chemistry. 2016; 18 :6046-6062. DOI: 10.1039/C6GC01531J - 120.
Bugg TD, Ahmad M, Hardiman EM, Singh R. The emerging role for bacteria in lignin degradation and bio-product formation. Current Opinion in Biotechnology. 2011; 22 :394-400. DOI: 10.1016/j.copbio.2010.10.009 - 121.
Antai SP, Crawford DL. Degradation of softwood, hardwood, and grass lignocelluloses by two streptomyces strains. Applied and Environmental Microbiology. 1981;42 :378-380. DOI: 10.1128/aem.42.2.378-380.1981 - 122.
Mycroft Z, Gomis M, Mines P, Law P, Bugg TDH. Biocatalytic conversion of lignin to aromatic dicarboxylic acids in Rhodococcus jostii RHA1 by re-routing aromatic degradation pathways. Green Chemistry. 2015; 17 :4974-4979. DOI: 10.1039/C5GC01347J - 123.
Sainsbury PD, Hardiman EM, Ahmad M, Otani H, Seghezzi N, Eltis LD, et al. Breaking down lignin to high-value chemicals: The conversion of lignocellulose to vanillin in a gene deletion mutant of Rhodococcus jostii RHA1. ACS Chemical Biology. 2013;8 :2151-2156. DOI: 10.1021/cb400505a - 124.
Khan MU, Ahring BK. Lignin degradation under anaerobic digestion: Influence of lignin modifications – A review. Biomass and Bioenergy. 2019; 128 :105325. DOI: 10.1016/j.biombioe.2019.105325 - 125.
Kato S, Chino K, Kamimura N, Masai E, Yumoto I, Kamagata Y. Methanogenic degradation of lignin-derived monoaromatic compounds by microbial enrichments from rice paddy field soil. Scientific Reports. 2015; 5 :14295. DOI: 10.1038/srep14295 - 126.
Duan J, Huo X, Du WJ, Liang JD, Wang DQ , Yang SC. Biodegradation of Kraft lignin by a newly isolated anaerobic bacterial strain, Acetoanaerobium sp. WJDL-Y2. Letters in Applied Microbiology. 2016;62 :55-62. DOI: 10.1111/lam.12508 - 127.
Hu M, Wang J, Gao Q , Bao J. Converting lignin derived phenolic aldehydes into microbial lipid by Trichosporon cutaneum. Journal of Biotechnology. 2018; 281 :81-86. DOI: 10.1016/j.jbiotec.2018.06.341 - 128.
Yaguchi A, Robinson A, Mihealsick E, Blenner M. Metabolism of aromatics by Trichosporon oleaginosus while remaining oleaginous. Microbial Cell Factories. 2017; 16 :206. DOI: 10.1186/s12934-017-0820-8 - 129.
Sànchez i Nogué V, Black BA, Kruger JS, Singer CA, Ramirez KJ, Reed ML, et al. Integrated diesel production from lignocellulosic sugars via oleaginous yeast. Green Chemistry. 2018;20 :4349-4365. DOI: 10.1039/C8GC01905C