Explanation of different cases.
Abstract
Metal foam as flow field for proton exchange membrane fuel cell (PEMFC) has demonstrated substantial promise for enhancing the distribution uniformities of reactants and temperature. In different types of PEMFC, however, the influence of metal foam flow field on cell performance is diverse. For liquid-cooled PEMFC, employing metal foam flow field encounters challenges in water removal. For air-cooled PEMFC, employing metal foam can help retain a certain amount of liquid water to avoid membrane dehydration. In an effort to further enhance the cell performance and practical application potential of metal foam in different types of PEMFC, the design strategy for metal foam flow field in PEMFCs is studied through this chapter. Experimental results revealed that for liquid-cooled PEMFC, the cathode side employing metal foam flow field raises the potential for water flooding and instability of operational voltage, which can be addressed through reasonably designing the structural characteristics of metal foam. Furthermore, the thermal management capability of air-cooled PEMFC can be boosted through adopting metal foam flow field, owing to the synergic improvement of forced convective heat transfer of reactant gas and electrochemical performance, which is the main factor of the thermal management improvement at high current.
Keywords
- PEMFC
- metal foam flow field
- design strategy
- water management
- thermal management
1. Introduction
Due to the global desire for more efficient and environmentally friendly energy usage, proton exchange membrane fuel cell (PEMFC), which is being recognized as a potential substitute for internal combustion engines, has gained increasing interest of scholars owing to its remarkable attributes such as excellent efficiency, low operating temperature, quickly starting speed, and environmentally clean operation [1]. The electrochemical mechanism and components of different types of PEMFC are depicted in Figure 1. In the past few years, PEMFC has experienced significant advancements and has found diverse applications in areas such as spacecraft, emergency supply, and transportation [2]. Enhancing the performance of PEMFC is crucial to expand its application areas.
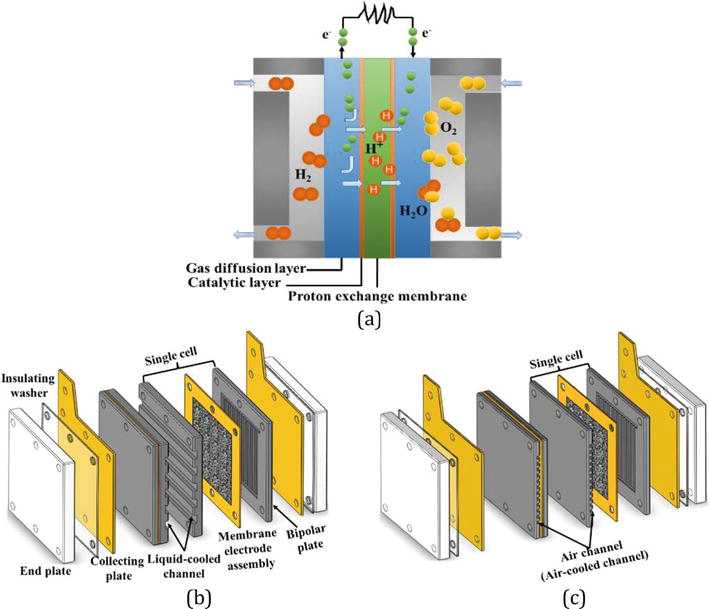
Figure 1.
The electrochemical mechanism and components of different types of PEMFC. (a) Schematics of the electrochemical mechanism of PEMFC. (b) Liquid-cooled PEMFC. (c) Air-cooled PEMFC.
Bipolar plate (BP), an essential constituent of PEMFC, plays a pivotal role in providing structural support and anchoring the membrane electrode assembly (MEA). Apart from these functions, flow field in BP performs additional vital functions, like distributing the reactants and products, collecting current and discharging, and so forth. The flow field design and optimization in BP play a crucial role in determining the performance of PEMFCs. Consequently, numerous scholars [3, 4, 5] have dedicated their efforts to designing and optimizing flow field for boosting the output performance of PEMFCs. Yan et al. [6] presented an innovative 3D serpentine flow channel that equips with a gradient wave structure. According to numerical and experimental results, in comparison with traditional serpentine flow channel, this innovative 3D serpentine flow channel exhibits the potential for enhancing the diffusion of the reactants to the catalytic layer (CL). Moreover, this innovative flow channel has the capability to augment the flow velocity, thus effectively removing excess liquid water. However, conventional flow field design in BPs frequently results in inhomogeneous gas distribution on the MEA particularly beneath the ribs. This, in result, leads to inhomogeneous electrochemical reaction and localized areas of elevated temperature at the MEA. The phenomenon of localized areas of elevated temperature is detrimental to the durability of the MEA. In recent times, there has been considerable interest in utilizing metal foam as flow distributor for PEMFC owing to its exceptional distribution properties.
Metal foam exhibits remarkable electrical and thermal conductive properties, in addition to possessing high porosity as well as a substantial specific surface area [7, 8]. The reactant distribution uniformity, thermal management, and performance of PEMFC can be effectively boosted by the adoption of metal foam flow field. The study conducted by Afshari et al. [9] involved a numerical comparison of the performances of PEMFCs equipped with a partially restricted flow field as well as metal foam flow field. The findings demonstrated that the distribution uniformities of current density and oxygen concentration at the CL can be boosted by the implementation of metal foam flow field. In addition, based on the experimental results of Awin and Dukhan [10], it was depicted that by comparing with serpentine flow field, metal foam design can offer more homogeneous distributions of temperature on the MEA. Nevertheless, for liquid-cooled PEMFC, the utilization of metal foam flow field causes water discharge issue affecting the operation stability. Tseng et al. [11] identified that some pores in metal foam were clogged by liquid water, causing the water flooding phenomenon. In contrast, in the case of air-cooled PEMFC, the adoption of a metal foam flow field demonstrates the capability to retain water leading to a better equilibrium between heat dispersion and water preservation. Kang et al. [12] carried out numerical and experimental researches to examine the influence of metal foam on the water management as well as output performance of air-cooled PEMFC. The findings obtained from their research revealed that the implementation of metal foam in the cathode side effectively inhibits the membrane dehydration phenomenon. In view of this, the design strategy for metal foam flow field in various types of PEMFC is crucial for improving the cell performance and enhancing the potential for practical implementation of metal foam flow field. However, the study related to the metal foam design strategy for PEMFC is rare in the open literature.
In this chapter, the design strategy for metal foam flow field in liquid-cooled PEMFC and air-cooled PEMFC is extensively investigated through three sections. The influence of the arrangement of metal foam flow field on the cell performance as well as water management is thoroughly explored. A 3-hour constant current density operational test is conducted for assessing the operation stability associated with the water flooding phenomenon. Structural characteristics of metal foam flow field are also developed for addressing the water flooding issue. Furthermore, the influence of metal foam flow field on the thermal management and performance of air-cooled PEMFC is studied.
2. Experimental
2.1 Design of metal foam flow field and assembled of single cell
The metal foam with various structures applied in this chapter was nickel-based (Guangjiayuan Electronic Materials Co., Ltd., Kunshan City). Since the active area of PEMFC is 50 mm × 50 mm, it is necessary to cut the sample by a laser with a precision of ±0.1 mm to guarantee that the pores located in the cutting region remain unclogged. Additionally, the experimental MEA adopted for this chapter was produced by Wuhan University of Technology. In Figure 2, the BP with different flow fields, MEA, and single cell used for experiments are depicted.
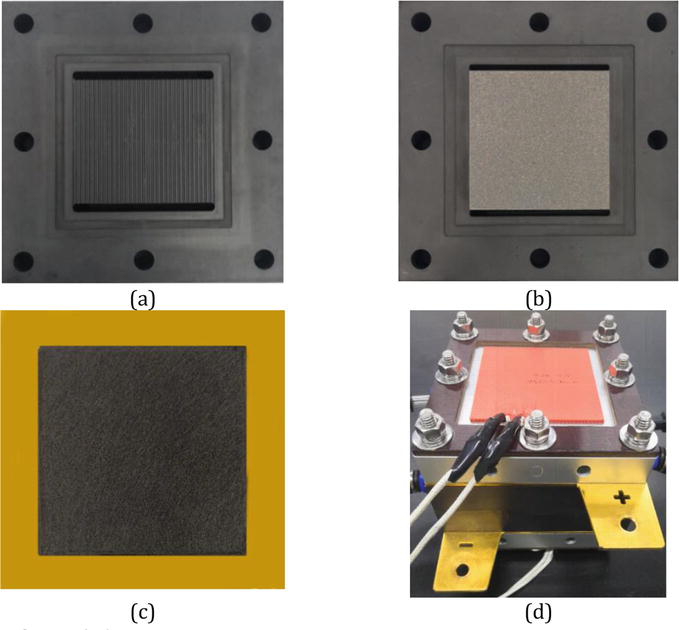
Figure 2.
Straight flow field, metal foam flow field, MEA, and single cell.
2.2 Test system
The experimental setup for testing PEMFC, referred to as the G60 fuel cell test system, is depicted in Figure 3. This system comprises various components like a gas storage device, heating device, pipeline network, moisture control device, thermal insulation device, monitoring and control device, PEMFC, reactants mass flow control device, and so on.
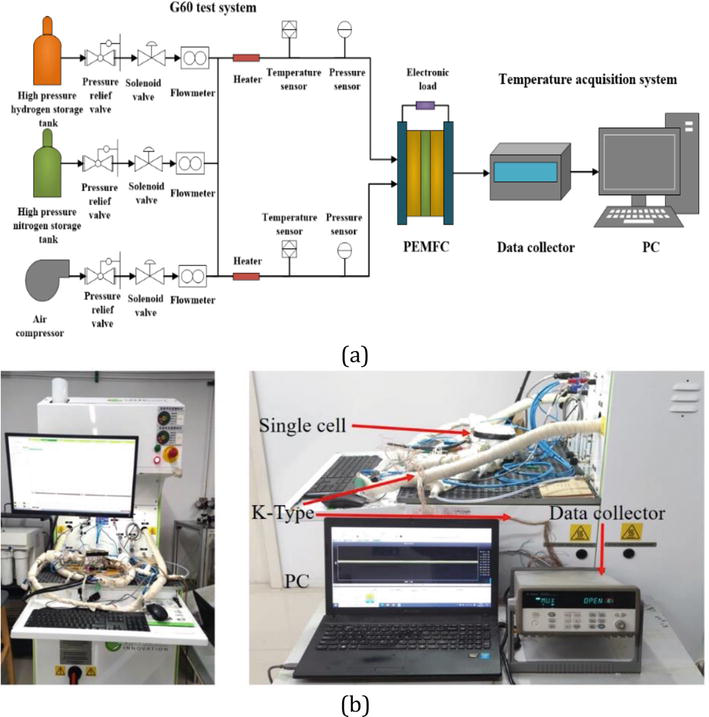
Figure 3.
Diagrammatic representation and picture of the entire experimental system.
3. Performance and water management investigation on the arrangement of metal foam flow field in liquid-cooled PEMFC
In this section, four cases are established as depicted in Table 1 to evaluate the influence of the arrangement of metal foam flow field on the performance of PEMFC. The difference in the water management of different cases is also examined. Note that the porosity, thickness, and pore diameter of metal foam employed in this section are 95–97%, 1, and 0.2 mm, respectively. The experimental operation conditions are also depicted in Table 2.
Case No. | Explanations |
---|---|
Case 1 | Straight flow field for anode and cathode |
Case 2 | Metal foam flow field for anode and straight flow field for cathode |
Case 3 | Straight flow field for anode and metal foam flow field for cathode |
Case 4 | Metal foam flow field for anode and cathode |
Table 1.
Operation condition | Value |
---|---|
Operation temperature | |
Relative humidity of | 0/0.5/0.8/1 |
Relative humidity of air | 0/0.5/0.8/1 |
Stoichiometric flow ratio of | 1.2 |
Stoichiometric flow ratio of air | 2.5 |
Operating pressure | 1 atm |
Table 2.
Operation conditions for experiments in this section.
3.1 Influence of relative humidity
The influence of relative humidity (RH) of reactants on the cell performance was studied in this subsection; Figure 4 shows the peak power density of four cases and the peak power density augmentation rate (
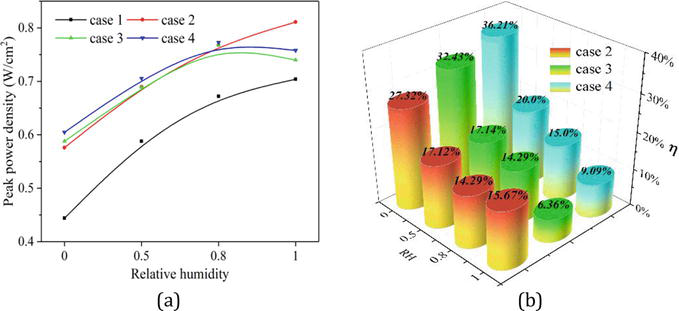
Figure 4.
(a) Peak power density of four cases under various RHs; (b) peak power density augmentation rate of cases 2–4 in comparison with that of case 1.
According to Figure 4, adopting metal foam flow field can result in the improved cell performance over traditional straight flow field, which conforms to the findings from Jo and Ju [13]. Additionally, when RH is set to 1, the augmentation rates of peak power density in cases 3 and 4, both utilizing metal foam flow field in the cathode side, are 6.36 and 9.09%, respectively. In case 2, the augmentation rate
3.2 Flooding test and analysis
According to the study conducted by Bao et al. [14], it was asserted that removing liquid water poses a greater challenge for metal foam flow field in comparison with traditional straight flow field, primarily owing to the complex internal structure impeding effective water removal. When RH and current are high, the implementation of metal foam in the cathode side results in the water flooding phenomenon, affecting the cell performance. In contrast, the implementation of metal foam flow field demonstrates water retention capability when RH is low, wetting the membrane, thereby enhancing the rate of mass transfer in the membrane and improving the cell performance in comparison with traditional straight flow field.
Considering the test processes mentioned above, PEMFC adopting metal foam as cathode flow field easily results in the occurrence of the water flooding phenomenon at high current and RH. In order to gain deeper insights into the flooding phenomenon, 3-hour constant current density operational test on four cases under different RHs was performed in this subsection. The current density is fixed at 1.5 A/cm2. Figure 5 presents the variations of voltage and current density over time in different cases under RH = 1. It is evident that over the 3 hours of operation, the voltages and current densities of cases 1 and 2 essentially remain steady. However, there were four minor voltage attenuations during the initial 105 minutes in cases 3 and 4. Following that, the voltage started to attenuate quickly. The underlying cause for this can be attributed to the implementation of metal foam as flow field in the cathode side in these two cases. In the course of PEMFC operation, liquid water slowly accrues and cannot be effectively removed in time, resulting in the reactants channel in the GDL to be filled. The voltage gradually decreased, while PEMFC operates in a constant current density model.
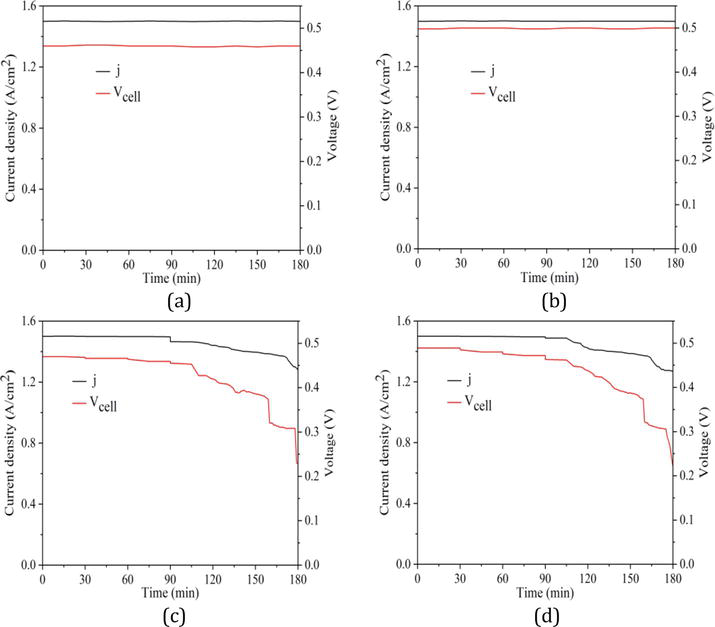
Figure 5.
Voltage and current density over time of different cases under RH of 1. (a) Case 1. (b) Case 2. (c) Case 3. (d) Case 4.
In Figure 6, the correlations between RH and crucial flooding time of cases 3 and 4 are depicted. It is evident that as RH decreases, the crucial flooding times for these two cases experience a gradual delay. Thus, it can draw the conclusion that arranging metal foam flow field in the cathode side leads to the water flooding issue, negatively impacting the cell performance.
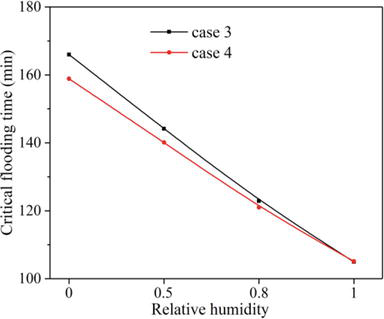
Figure 6.
Correlations between the RH and crucial time of flooding in cases 3 and 4.
4. Investigation of water management and structure optimization of metal foam flow field in liquid-cooled PEMFC
As stated before, the water flooding phenomenon occurs under the high current density and high RH, when metal foam was adopted in the cathode side of PEMFC. In addition, Bao et al. [15] pointed out that the reactant velocity affected by structural parameters of flow field has a significant influence on the water discharging in PEMFC. Therefore, the influences of the structural characteristics of metal foam like compression rate and pore per inch (PPI) on the water management as well as cell performance are investigated in this section. It should be noted that the cathode flow field depth is 0.5 mm. Traditional straight flow field is set with channel dimensions of 1 mm for width, height, and rib width in the anode side. RHs for both anode and cathode are all 1.
4.1 Influence of compression rate
For the purpose of examining the influence of the metal foam compression rate on the water management and cell performance, metal foam flow fields with various compression rates are set up in the cathode side of PEMFC in this subsection. Figure 7 displays SEM images of metal foam including various compression rates. Obviously, the pore diameter of metal foam in the direction perpendicular to the reactants flow reduces, while it basically remains unchanged in the flow direction of reactants as the increase of the compression rate. Hence, the reactant diffusion to the GDL can be enhanced through the compression of metal foam. However, compressing metal foam will also lead to a reduction in porosity. Based on the results of Shin et al. [16] and Tseng et al. [11], metal foam has a larger porosity, which indicates that there is more space for reactants improving the homogeneity of the reactant distribution. Thus, compressing metal foam does not favor achieving homogeneous reactant distribution.
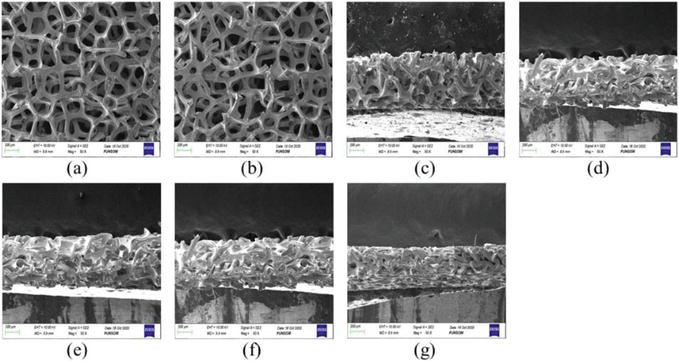
Figure 7.
SEM images: (a, b) overhead perspective of metal foam exhibiting compression rates of 0 and 0.75; (c, g) cross-sectional depiction of metal foams showcasing diverse compression rates, specifically 0, 0.38, 0.50, 0.69, and 0.75.
From Figure 8(a), when the compression rate of metal foam flow field in the cathode side rises, it is clear that the cell performance is enhanced. As shown in Figure 8(b), the cell performance enhancement also improves the air utilization. The reason is that reactant diffusion to the CL is boosted by the increment in the compression rate of metal foam flow field. This notion is also supported by the findings of Park et al. [17]. More crucially, as demonstrated in Figure 8(c), the increment in the compression rate also leads to better voltage stability of PEMFC, suggesting that the water management capability is enhanced. It is worth noting that the operation voltage is totally stable as soon as the metal foam compression rate rises over 0.69. In order to analyze this point more clearly, the duration of constant current density operational tests for PEMFCs utilizing the compression rates of 0.69 and 0.75 was extended to 9 hours as depicted in Figure 8(d). Based on the results presented in Figure 8
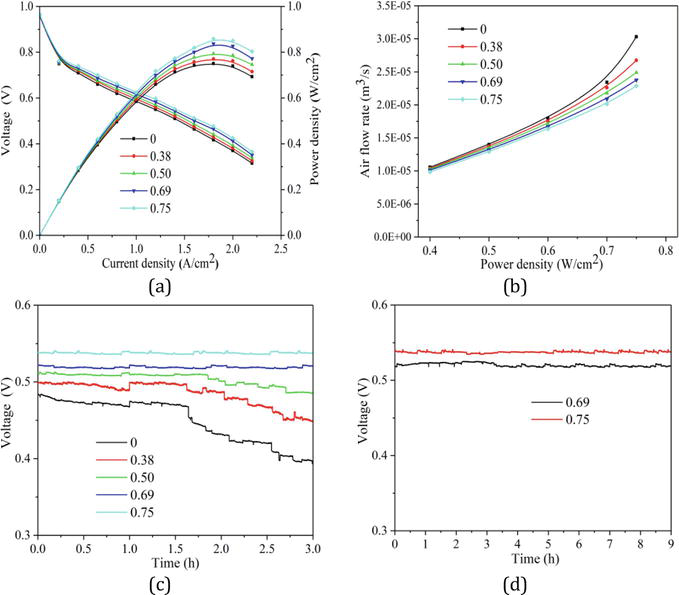
Figure 8.
(a) Polarization curves; (b) comparison of air flow rate; (c, d) voltage over time.
4.2 Influence of PPI
In this subsection, the cathode of PEMFC is equipped with metal foam flow fields featuring various PPIs. From Figure 9, the pore density rises as the increment of PPI of metal foam, which is helpful for the reactants to be distributed uniformly. However, the increase of PPI will also lead to a reduction in pore diameter and permeability of metal foam. Liquid water first percolates into metal foam flow field
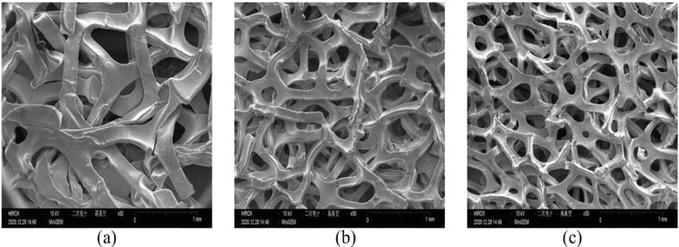
Figure 9.
SEM images: Overhead perspective of metal foams with various PPIs: (a) 35 PPI, (b) 75 PPI, (c) 110 PPI.
The polarization curves of PEMFCs comprising various PPIs in the cathode side are depicted in Figure 10(a). It is interesting to observe that the performance of PEMFC adopting metal foam flow field with 75 PPI is the best. This outcome can be attributed to the synergistic influence between reactant distribution and water management. In Figure 10
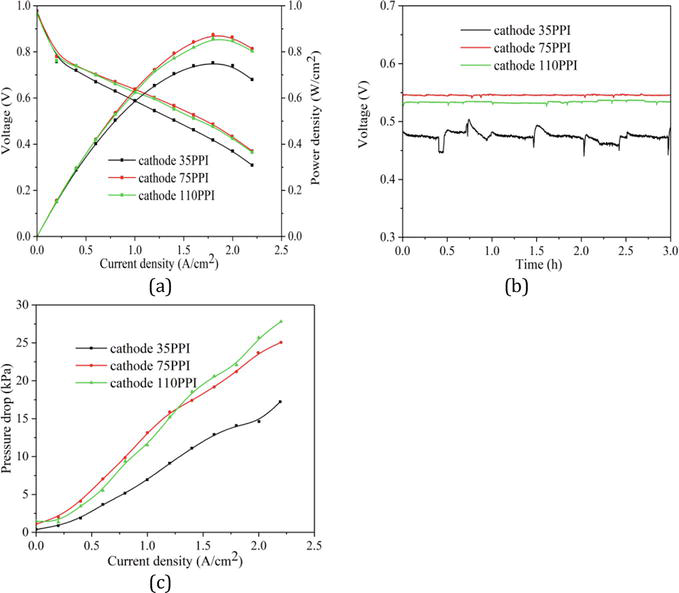
Figure 10.
(a) Polarization curves; (b) voltage over time; (c) pressure drops from cathode inlet to outlet under different current densities.
5. Thermal management enhancement of air-cooled PEMFC through the implementation of metal foam flow field
In this section, the influence of metal foam flow field on the thermal management and performance of air-cooled PEMFC is examined, considering the superior heat dissipation and water retention characteristics of metal foam. Straight flow field is implemented for the anode side, the channel sizes of which are identical to those mentioned in the preceding sections. Table 3 provides a comprehensive list of the geometric parameters related to the cathode flow field. In addition, the experimental operation conditions are also provided in Table 4.
Case No. | Geometric parameters of cathode flow field | Flow field type | |
---|---|---|---|
Length/width/height of channel (mm) | Width of rib (mm) | ||
Case 5 | 50/1/1 | 1 | Straight flow field |
Case 6 | 50/1/1 | 1 | Metal foam flow field |
Case 7 | 50/3/1 | 1 | Straight flow field |
Case 8 | 50/3/1 | 1 | Metal foam flow field |
Case 9 | 50/5/1 | 1 | Straight flow field |
Case 10 | 50/5/1 | 1 | Metal foam flow field |
Case 11 | 50/5/2 | 1 | Metal foam flow field |
Case 12 | 50/5/3 | 1 | Metal foam flow field |
Table 3.
Descriptions of experiment cases in this section.
Operation condition | Value |
---|---|
Inlet temperature of | |
Inlet temperature of air | |
Relative humidity of inlet | 0% |
Relative humidity of inlet air | 50% |
Stoichiometric flow ratio of | 1.5 |
Stoichiometric flow ratio of air | 50 |
Operating pressure (gauge pressure) | 1 atm |
Table 4.
Operation conditions for the experiments in this section.
5.1 Thermal performance evaluation
As air-cooled PEMFC operates, electricity (
where
The temperature acquisition system was adopted to gather the temperature information of PEMFC. A total of 20 temperature detection points were arranged as depicted in Figure 11. The mean cell temperature is defined as the average temperature of 20 thermocouples. The maximum temperature difference (
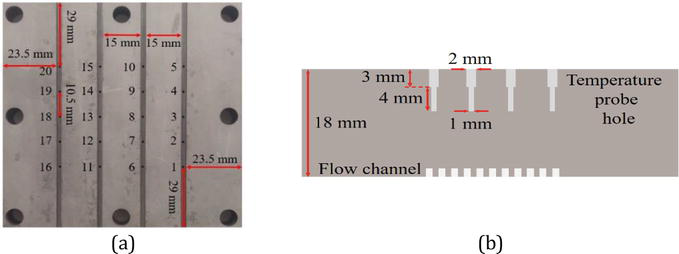
Figure 11.
Exact locations of thermocouples.
Note that
5.2 Influence of metal foam flow field on thermal management of air-cooled PEMFC
In this subsection, two cases, namely, cases 5 and 6, are chosen to examine the influence of metal foam flow field on the thermal management of air-cooled PEMFC. According to the data from Figure 12
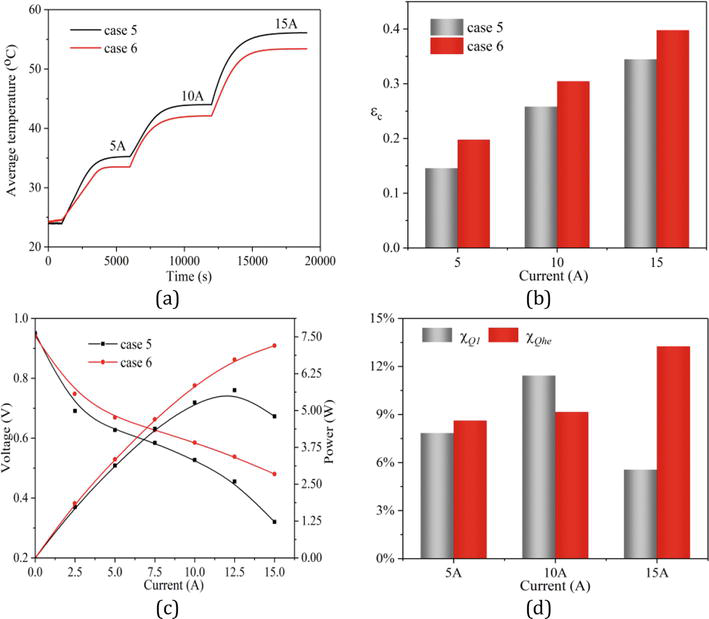
Figure 12.
(a) Cell temperature over time; (b) comparison of the cooling efficiency; (c) polarization curves; (d) variation rates of
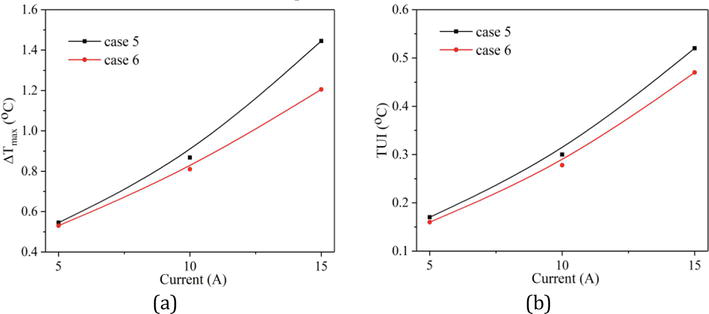
Figure 13.
(a, b) temperature uniformities of different cases.
where
5.3 Influence of width of metal foam flow field
In this subsection, the study focused on investigating the influence of the width of metal foam on the thermal management of air-cooled PEMFC. Cases 6, 8, and 10 are analyzed. Figure 14
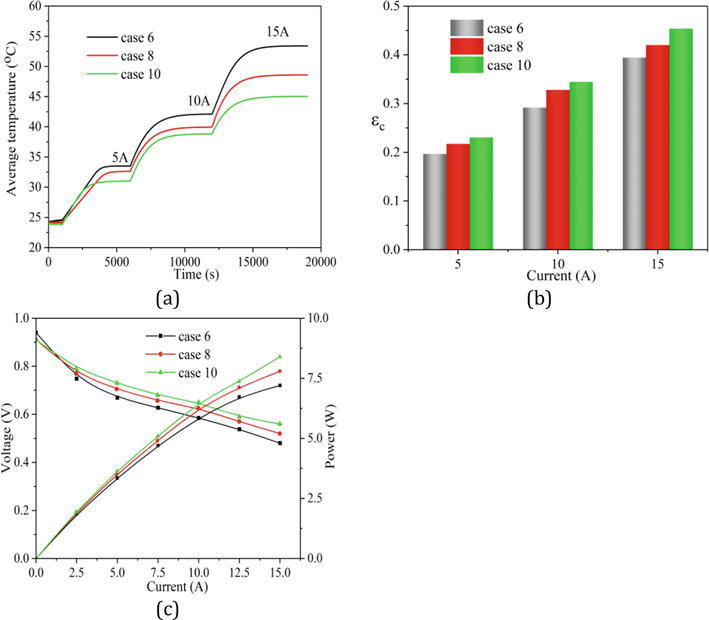
Figure 14.
(a) Cell temperature over time; (b) comparison of the cooling efficiency; (c) polarization curves.
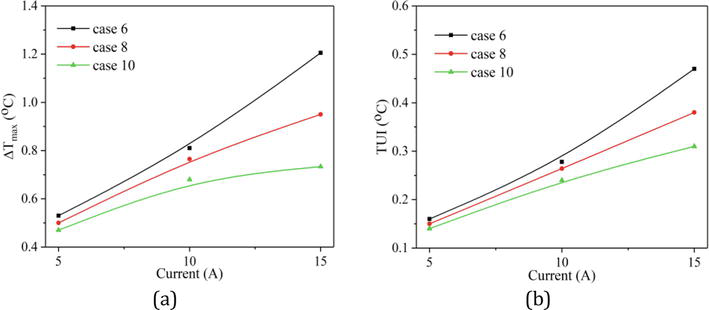
Figure 15.
(a, b) temperature uniformities of different cases.
5.4 Influence of height of metal foam flow field
In this subsection, cases 10, 11, and 12 are chosen for analyzing the influence of the height of metal foam on the thermal management of air-cooled PEMFC. Observing from Figure 16
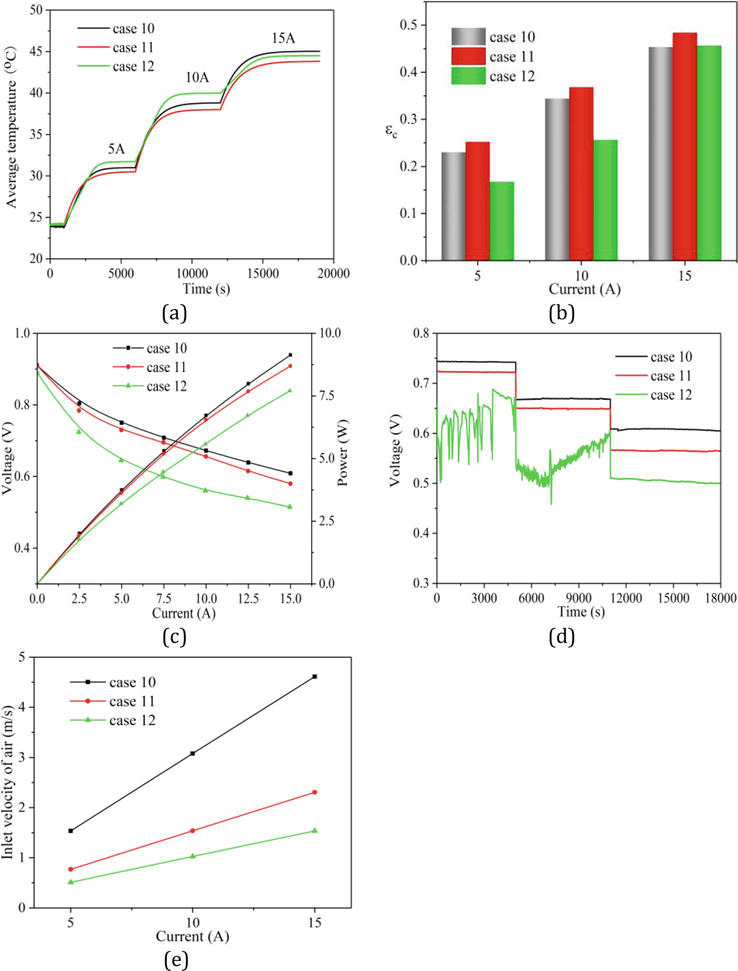
Figure 16.
(a) Cell temperature over time; (b) comparison of the cooling efficiency; (c) polarization curves; (d) voltage over time; (e) air inlet velocities of three cases.
6. Conclusion
This chapter focuses on the experimental investigation of the design strategy for metal foam flow field in different types of PEMFC. The influence of the arrangement of metal foam flow field on the performance and water management of liquid-cooled PEMFC was experimentally examined. Additionally, the structural characteristics of metal foam were optimized to enhance the water management and cell performance. Furthermore, in air-cooled PEMFC, the influence of metal foam flow field on the thermal management was also explored. On the basis of the exhausted experiments, the following conclusions have been drawn:
Under low RH, metal foam flow field demonstrates the capability to retain water, which is essential for wetting the membrane and enhancing the cell performance. Conversely, under high RH, employing metal foam flow field in the cathode side increases the possibility of water flooding, thereby impacting the liquid-cooled PEMFC performance negatively. Interestingly, when only the anode side utilizes metal foam flow field, there is no instance of the water flooding phenomenon, leading to an improved cell performance.
Under the operating conditions with complete relative humidity and a constant current density of 1.5 A/cm2, liquid-cooled PEMFC utilizing traditional straight flow field in the cathode side demonstrates steady current density and voltage throughout a 3-hour operation. However, when utilizing metal foam flow field in the cathode side, PEMFC experienced slight flooding within 105 minutes, leading to a slight degradation in voltage. Subsequently, the voltage decayed quickly. Furthermore, as relative humidity decreases, the crucial flooding times are gradually delayed.
Performance enhancement in liquid-cooled PEMFCs is observed with an increase in metal foam compression rate in the cathode side. Additionally, once the compression rate in the cathode side exceeds 0.69, the operation voltage demonstrates complete stability during 3-hour operation.
Regarding PPI of metal foam, it is intriguing to observe that optimal PPI, namely, 75, is obtained for the cathode side. This is attributed to the synergistic influence between the water management and gas distribution of metal foam flow fields with various PPIs.
The implementation of metal foam as cathode flow field for air-cooled PEMFC can effectively enhance its thermal management capability. Moreover, the implementation of metal foam can help even out the temperature distribution owing to its mixing effect for reactants.
Enhancing the width of metal foam results in better thermal management for air-cooled PEMFC. In the case where the height is fixed at 1 mm and the width rises from 1 to 5 mm, the cell temperature lowers by 8.4°C, accompanied by a 14.7% increase in the cooling efficiency at current of 15 A.
When the width of metal foam flow field is fixed at 5 mm, raising the height of metal foam from 1 to 2 mm results in a slight improvement in the cooling efficiency of air-cooled PEMFC. However, a further increase in the height to 3 mm leads to a minor water flooding phenomenon due to the low inlet velocity under current below 10 A.
Acknowledgments
This work is supported by National Natural Science Foundation of China (Nos. 52276184, 52176062, 51976055, 52076072) and the Science and Technology Innovation Program of Hunan Province (2020RC4040, 2021GK2017).
Nomenclature
Qele | electric energy (W) |
Qhe | heat (W) |
Q1 | forced convective heat transfer (W) |
Q2 | cell temperature rise (°C) |
I | current (A) |
V | voltage (V) |
Er | the theoretical reversible voltage (V) |
ncell | number of cells (−) |
qair | flow rate of air (m3/s) |
T | temperatures (K) |
ΔTini | initial temperature difference (°C) |
TUI | temperature uniformity index (°C) |
cp | specific heat of air (J/(kg⋅K)) |
Subscripts and superscripts
max | maximum |
min | minimum |
ave | average |
ini | initial |
he | heat |
in | inlet |
out | outlet |
References
- 1.
Barelli L, Bidini G, Ottaviano A. Optimization of a PEMFC/battery pack power system for a bus application. Applied Energy. 2012; 97 :777-784. DOI: 10.1016/j.apenergy.2011.11.043 - 2.
Kang S, Min K. Dynamic simulation of a fuel cell hybrid vehicle during the federal test procedure-75 driving cycle. Applied Energy. 2016; 161 :181-196. DOI: 10.1016/j.apenergy.2015.09.093 - 3.
Wan Z, Quan W, Yang C, Yan H, Chen X, Huang TM, et al. Optimal design of a novel M-like channel in bipolar plates of proton exchange membrane fuel cell based on minimum entropy generation. Energy Conversion and Management. 2020; 205 :112386. DOI: 10.1016/j.enconman.2019.112386 - 4.
Cai G, Liang Y, Liu Z, Liu W. Design and optimization of bio-inspired wave-like channel for a PEM fuel cell applying genetic algorithm. Energy. 2020; 192 :116670. DOI: 10.1016/j.energy.2019.116670 - 5.
Chen H, Guo H, Ye F, Ma CF. Modification of the two-fluid model and experimental study of proton exchange membrane fuel cells with baffled flow channels. Energy Conversion and Management. 2019; 195 :972-988. DOI: 10.1016/j.enconman.2019.05.071 - 6.
Yan X, Guan C, Zhang Y, Jiang K, Wei G, Cheng X, et al. Flow field design with 3D geometry for proton exchange membrane fuel cells. Applied Thermal Engineering. 2019; 147 :1107-1114. DOI: 10.1016/j.applthermaleng.2018.09.110 - 7.
Yang C, Nakayama A. A synthesis of tortuosity and dispersion in effective thermal conductivity of porous media. International Journal of Heat and Mass Transfer. 2010; 53 :3222-3230. DOI: 10.1016/j.ijheatmasstransfer.2010.03.004 - 8.
Nakayama A, Ando K, Yang C, Sano Y, Kuwahara F, Liu J. A study on interstitial heat transfer in consolidated and unconsolidated porous media. Heat and Mass Transfer. 2009; 45 (11):1365-1372. DOI: 10.1007/s00231-009-0513-x - 9.
Afshari E, Mosharaf-Dehkordi M, Rajabian H. An investigation of the PEM fuel cells performance with partially restricted cathode floe channels and metal foam as a flow distributor. Energy. 2017; 118 :705-715. DOI: 10.1016/j.energy.2016.10.101 - 10.
Awin Y, Dukhan N. Experimental performance assessment of metal-foam flow fields for proton exchange membrane fuel cells. Applied Energy. 2019; 252 :113458. DOI: 10.1016/j.apenergy.2019.113458 - 11.
Tseng CJ, Tsai BT, Liu ZS, Cheng TC, Chang WC, Lo SK. A PEM fuel cell with metal foam as flow distributor. Energy Conversion and Management. 2012; 62 :14-21. DOI: 10.1016/j.enconman.2012.03.018 - 12.
Kang DG, Park C, Lim IS, Choi SH, Lee DK, Kim MS. Performance enhancement of air-cooled open cathode polymer electrolyte membrane fuel cell with inserting metal foam in the cathode side. International Journal of Hydrogen Energy. 2020; 45 :27622-27631. DOI: 10.1016/j.ijhydene.2020.07.102 - 13.
Jo A, Ju H. Numerical study on applicability of metal foam as flow distributor in polymer electrolyte fuel cells (PEFCs). International Journal of Hydrogen Energy. 2018; 43 (30):14012-14026. DOI: 10.1016/j.ijhydene.2018.01.003 - 14.
Bao Z, Niu Z, Jiao K. Numerical simulation for metal foam two-phase flow field of proton exchange membrane fuel cell. International Journal of Hydrogen Energy. 2019; 44 (12):6229-6244. DOI: 10.1016/j.ijhydene.2019.01.086 - 15.
Bao Z, Wang Y, Jiao K. Liquid droplet detachment and dispersion in metal foam flow field of polymer electrolyte membrane fuel cell. Journal of Power Sources. 2020; 480 :229150. DOI: 10.1016/j.jpowsour.2020.229150 - 16.
Shin DK, Yoo JH, Kang DG, Kim MS. Effect of cell size in metal foam inserted to the air channel of polymer electrolyte membrane fuel cell for high performance. Renewable Energy. 2018; 115 :663-675. DOI: 10.1016/j.renene.2017.08.085 - 17.
Park JE, Hwang WC, Lim MS, Kim SJ, Ahn CY, Kim OH, et al. Achieving breakthrough performance caused by optimized metal foam flow field in fuel cells. International Journal of Hydrogen Energy. 2019; 44 :22074-22084. DOI: 10.1016/j.ijhydene.2019.06.073 - 18.
Zhao J, Huang ZP, Jian BX, Bai XY, Jian QF. Thermal performance enhancement of air-cooled proton exchange membrane fuel cells by vapor chambers. Energy Conversion and Management. 2020; 213 :112830. DOI: 10.1016/j.enconman.2020.112830 - 19.
Mohamed WANW, Talib SFA, Zakaria IA, Mamat AMI, Duad WRW. Effect of dynamic load on the temperature profiles and cooling response time of a proton exchange membrane fuel cell. Journal of the Energy Institute. 2018; 91 (3):349-357. DOI: 10.1016/j.joei.2017.02.006 - 20.
Peng M, Chen L, Zhang RY, Xu WQ, Tao WQ. Improvement of thermal and water management of air-cooled polymer electrolyte membrane fuel cells by adding porous media into the cathode gas channel. Electrochimica Acta. 2022; 412 :140154. DOI: 10.1016/j.electacta.2022.140154