Abstract
Proton therapy is an effective and safe method to treat tumors in human body. Instead of conventional radiation (X-rays), this technique uses a heavy charged particles (protons) to treat cancer. This chapter reviews the basic aspects of the physics of proton therapy, including proton beam properties, proton interaction mechanisms, and radiation effects induced in the human tissue. A more highly conformal technique of proton therapy called “pencil beam scanning”, based on intensity-modulated proton therapy (IMPT), will be also developed. The uncertainty in the determination of the relative biological effectiveness (RBE) will also be discussed in light of recent experimental results. We conclude the chapter by discussing future developments and potential challenges of proton therapy.
Keywords
- proton beam
- interaction mechanism
- energy loss
- dose-depth distribution
- Bragg peak
- proton therapy
1. Introduction
Following Robert Wilson’s breakthrough publication “Radiological Use of Fast Protons” in 1946, which revolutionized the field of radiotherapy, proton therapy became a modality with increasing importance. Since the first treatments in the 1950s, proton beam therapy (PBT) has been increasingly used in hospital settings with similar workflows to conventional radiotherapy. Nearly 200,000 patients underwent proton beam treatment worldwide in 2020. The number of proton therapy (PT) installations will soon reach one hundred. Figure 1 shows the increasing current treatment rooms open worldwide and those expected to open in the next few years.
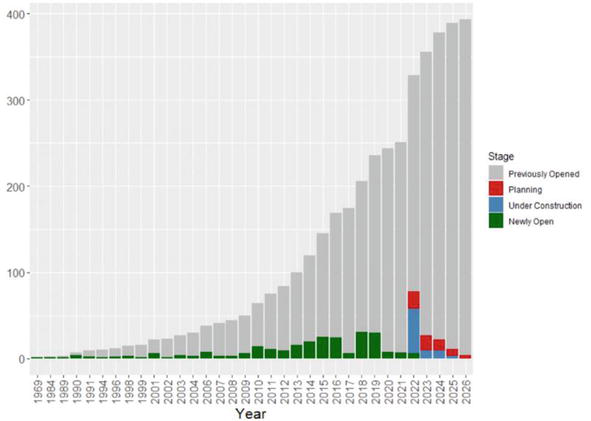
Figure 1.
Cumulative number of proton treatment rooms opened since 1964 and those projected until 2026 [
Due to the favorable physical properties of protons, the use of PBT has constantly increased over the last decade. The international scientific community has made great efforts to improve the performance and effectiveness of this technology. The aim of this chapter was to report the characteristics and current developments in PBT.
2. Physical aspects
2.1 Protons interactions
When a beam of protons passes into homogeneous matter, it undergoes successive interactions with atomic electrons and nucleus. First, protons lose continuously their kinetic energy through frequent inelastic Coulomb interactions with atomic electrons (excitation and ionization of atoms). Despite these interactions, protons travel in a nearly straight line as their rest mass is much higher than that of electron (1832 times). Seeing their energy decreased, protons experience a repulsive elastic Coulombic interaction with the atomic nucleus. Due to its large mass, nucleus deflects the proton from its original straight line path. On the other hand, the non-elastic nuclear reactions between protons and the target atomic nucleus are less likely. Proton Bremsstrahlung is theoretically possible, but, at therapeutic proton beam energies, this effect is also negligible.
2.2 Protons energy loss
The energy loss of incoming protons due to inelastic Coulombic interactions with atomic electrons in the irradiated medium is described by the linear stopping power
or frequently the mass stopping power
where
At lower energy (velocity), so in the end of its range, the proton interacts with irradiated medium through nuclear interactions. The main damage thus produced in the target is due to these interactions and it occurs in the end of proton range. This distribution of energy deposition, markedly different from that of conventional radiations, is useful for radiotherapy, as the overwhelming majority of proton energy is deposited at the end of the proton range.
We define also the Linear energy transfer (LET) as the ratio of energy transferred by a charged particle (dE) to the target atoms along its path through tissue (dx). In other words, LET is a measure of the density of ionizations along a radiation beam:
In proton therapy, we used commonly the absorbed dose which can be calculated by the relation (4). For proton beam fluence Φ in proton/cm2 and S/ρ in MeV/(g/cm2), dose is calculated as:
2.3 Proton range
The trajectory of most protons in matter is almost linear. Therefore, the path length of the proton is almost equal to its projected range, which is an excellent approximation of range in most clinical situations. In this case, the range (
2.4 Dose-depth distribution
Plotting the absorbed radiation dose versus the penetration depth of the protons, the emerged distribution is called Bragg distribution or Bragg curve (Figure 2).
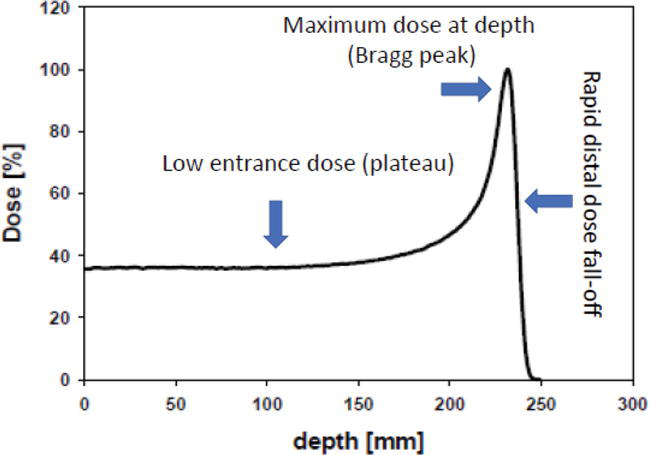
Figure 2.
Typical dose deposition as a function of depth for a proton beam.
As can be seen from the figure, the depth-dose curve for protons is characterized firstly by a low dose plateau, in the entrance region, caused predominantly by the slowly increasing energy loss with decreasing proton energy. The low absorbed energy in this region is due mainly to the electronic excitation and ionization of the target atoms. At a critical depth, the dose-depth curve experiences a distal rapid dose build-up, culminating in a maximum, and a steep fall-off at the end of their range. This behavior displayed by protons beam at the end of their path is called “Bragg peak”.
The drastic increase of the absorbed dose occurs at low energies (velocity) of the protons, in which the nuclear interactions are predominates. In this region of few millimeters, the protons deliver the majority of their energy at the target and produce the main damage. The relative height of this “Bragg peak” is about 3–4 times the absorbed dose in the plateau.
It is worth noting that the distal fall-off in the end of the Bragg curve is very steep. In the case of 200 MeV proton beam, the fall of the peak dose from 90 to 10% occurs in about 7 mm. The depth dose distribution displayed by proton, especially the distal fall-off in the end of the dose-depth curve, has made the proton as a real candidate for the treatment of tumors in the human body. This behavior is in line with the requirement of radiation therapy, in which researches seek to reduce the absorbed dose during the proton path in order to preserve the healthy tissues and deliver almost all of it in the tumor area. Therefore, protons can deliver a high dose to the tumor volume while sparing surrounding healthy tissues and organs at risk.
In proton beam therapy, the energetic proton travels the human tissue so fast, almost at two-thirds the speed of light, that the number of interactions in each millimeter that it travels is relatively low. So, the radiation dose absorbed by healthy tissues is relatively low. But after millions of interactions (electronic interactions), the proton will gradually slow down and stop. At the end of its path, when its speed is considerably reduced, the proton undergoes many more interactions (nuclear interactions) in a few millimeters where it deposits the majority of its energy. In this region of tumor volume (10–20 mm), the sharp energy loss results in a dramatical increase in radiation dose (high dose peak) at the end of the proton path (Bragg peak).
By varying the energy of proton beam, the Bragg peak can be moved to different depths within the patient. Knowing the Dose-depth distribution, we can know the dose maximum at the position of the tumor.
The depth of the Bragg peak is determined by the initial energy of the beam. As a prominent example of the proposed theories, Bortfeld [3] modeled the Bragg curve based on the power-law approximation where the range R0 of protons in the medium is related to their initial energy E0:
For proton energies used in therapy (
In radiotherapy, a lot of time is spent planning each treatment to ensure that the appropriate energy and dose are delivered to the tumor while minimizing the dose to normal organs as much as possible. This challenge is raised by proton therapy which has featured this particle as an appropriate candidate for radiation therapy.
3. Straggling
If all protons would lose the same amount of energy per scattering event, all would display the same range. However, the interaction of these charged particles with matter must be considered as a statistical process. Consequently, monoenergetic protons passing through matter will lose slightly different amounts of energy and will not all stop at precisely the same depth. This statistical fluctuation in the energy loss results in an uncertainty in the particle range, called “range straggling” (Figure 3).
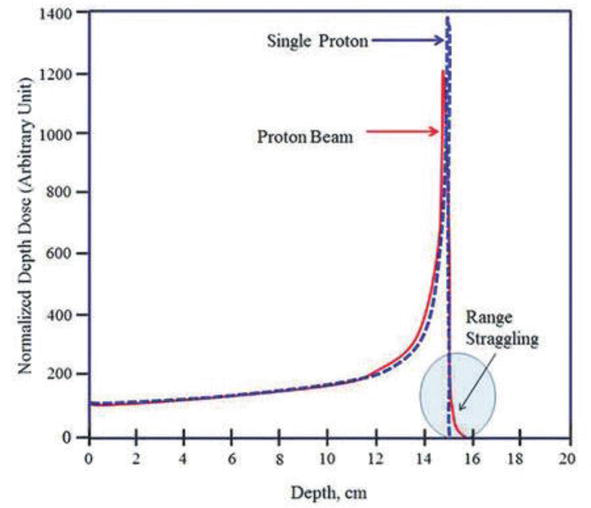
Figure 3.
Depth-dose curve of a proton beam, showing the Bragg peak and range straggling [
4. PBT and conventional radiations therapy
Photon therapy and proton therapy are the two main kinds of radiation therapy used in the treatment of cancer. These are different forms of ionizing radiation that work by damaging cancer cells so that they cannot reproduce and possibly die. While photons release energy along the entire path, they travel in the body, protons reserve most of energy during their path and release it where they stop in the tumor (peak Bragg) (Figure 2). This fundamental difference allows the proton to treat the tumor while minimizing radiation exposure to the rest of the body. Protons therefore have dosimetric characteristics different from those of conventional radiation used in radiotherapy. As indicated by Figure 4, after a short expansion of the absorbed dose, conventional radiation exhibits exponentially decreasing energy loss with increasing depth in medium.
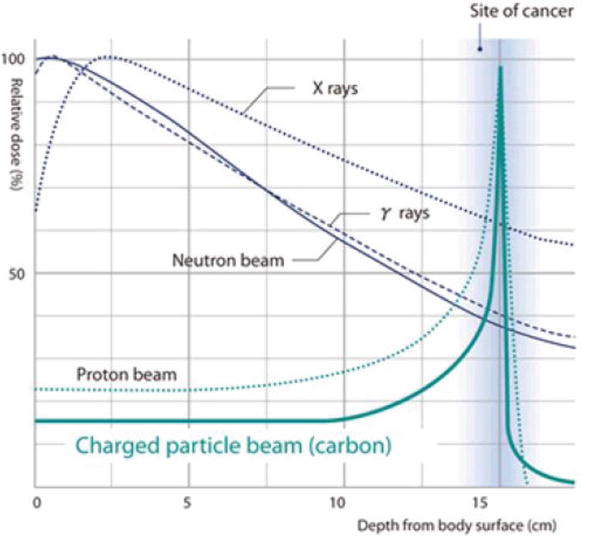
Figure 4.
Depth-dose curve of a proton, carbon ion, and other conventional radiations.
In contrast, protons exhibit a plateau of low entrance dose followed by a drastic increase in energy deposition with penetration depth leading to a maximum “Bragg peak” followed by a rapid distal dose fall-off at the end of the proton range (Figure 4).
This magical evolution of proton energy loss with the penetration depth in matter is consistent with the requirements of radiotherapy. Indeed, effective and safe treatment of tumors inside the human body requires two conditions: sparing of normal tissue that separates the body surface from the tumor and ensures that almost all of the energy will be deposited in the tumor area. It is clear that these requirements are satisfied in the dose-depth distribution of the protons (Figure 4), which deliver a very low dose in the first phase of their path then release almost all of their energy at the end of their path in a well-defined position.
Another important advantage of the proton radiotherapy over to conventional radiation is that protons finally stop in matter, and the dose beyond the stopping position is negligible. Due to this finite range in tissue, the delivered dose in the proton radiation therapy is more sensitive to density changes, when compared to external beam photon radiation therapy. In summary, most of radiation energy of photons is deposited outside the target, while most of radiation energy of protons is deposited inside the target.
On the other hand, we know that tumors are damaged after a series of interactions between the projectile and the cancer leading to DNA breaking. They are unable to repair or copy themselves, and therefore they will die. In this way, many experts believe that protons are more successful at breaking DNA than photons [5].
5. Problems and challenges of proton therapy
So far, we have seen that protons are best suited for treating cancer with radiotherapy. But, if we consider the local extension of the tumor, proton therapy, as presented above, cannot be the suitable approach for such treatment. However, a full understanding of proton-matter interactions and a dose-depth distribution allows us to solve the two main physical problems that may arise in proton therapy: designing proton beam according to the tumor shape, and predicting the dose distribution in human tissue. To handle the mentioned problems, proton beam energy could be adjusted to reach tumor position (beam range) and shaped to conform to the tumor area. The last one is accomplished by the modulation width of the spread-out Bragg peak (SOBP).
5.1 Spread-out Bragg peak (SOBP)
The Bragg peak of monoenergetic proton beam is too narrow to cover extent of tumor volume. To produce wider depth coverage, the location of the Bragg peak can be expanded as needed using proton beams of different energies, resulting in the spread-out Bragg peak (SOBP). The SOBP is produced by superimposing Bragg peaks of different proton energies, and thereby obtaining a widened region of homogeneous dose [6, 7, 8]. This energy degrader is suitable for treating tumors at any depth (Figure 5).
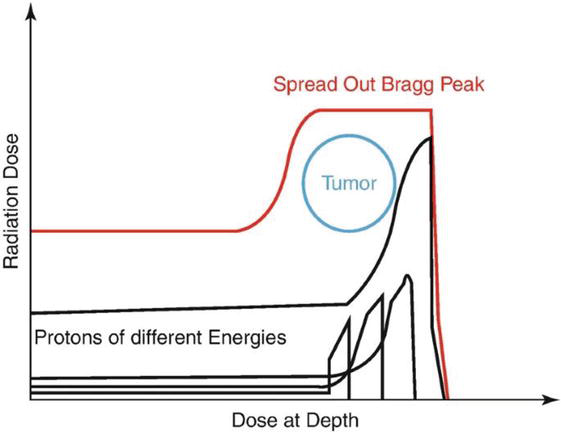
Figure 5.
Superimposition of Bragg peaks of different energies to have spread-out Bragg peak (SOBP).
For spreading the Bragg peak, proton therapy is generally administered through two different beam delivery systems: scattering and scanning beam delivery [9].
5.1.1 Passive scattering systems
In passive scattering mode, the narrow proton beam is laterally broadened by a scattering device and a modulator is used to generate the SOBP (Figure 6). A metallic filter is used to generate beam spreading with inverse square fall-off of intensity. It is then shaped using a second scatterer and collimator to provide a uniform field at the target volume. The disadvantages of this mode are significant dose delivered along the entrance path, extremely sensitive to the movements of target, and minimized integral dose.
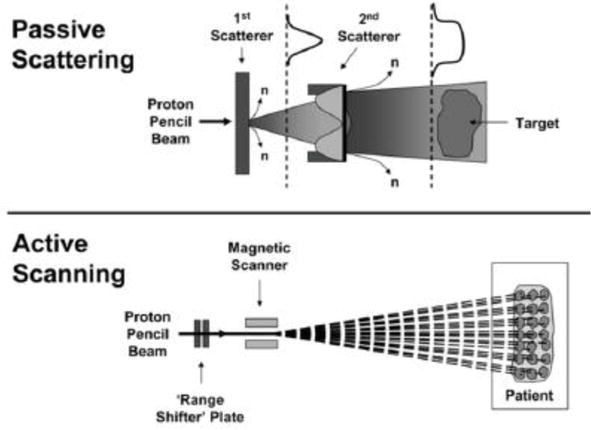
Figure 6.
Passive scattering and active scanning modes.
5.1.2 Active scanning systems (pencil beam scanning)
Pencil beam scanning (the beam roughly resembles a pencil) is a more conformal proton therapy technique which is now increasingly sought after in hospitals because it allows better conformation of the absorbed dose to the tumor. Compared to other proton beam methods, this technique allows the proton beam to be directed so precisely to the exact desired position in the tumor where the protons release their maximum energy dose, whereas, a lower dose is delivered to adjacent healthy tissue. Pencil beam scanning uses magnets to steer the proton beam, creating a customized, three-dimensional delivery shape. The target is divided into layers that are positioned at a user-defined interval. During treatment, the dose is deposited layer by layer, from the most distal layer to the most proximal layer sequentially. Within each layer, the dose is deposited through individual spots that can have unique intensities and positions (Figure 6). Therefore, the dose is conformed to the specific shape of the tumor and destroys cancer cells while preserving critical structures nearby.
In this technique, the material present in the beam path is minimized, which will reduce beam losses and the generation of unwanted secondary particles.
The active scattering technique or pencil beam scanning has two major advantages over the passive scattering technique. First, it allows for shaping of both proximal and distal edges of the treatment field by decreasing the entry dose while maintaining absence of exit dose. Second, the neutron scatter, which is of concern regarding secondary cancer induction, is significantly reduced. On top of that, pencil beam scanning introduces the possibility of intensity-modulated proton therapy (IMPT) [10, 11].
In Figure 7, a narrow pencil beam of high energy ≈250 MeV at the entrance, produced by a cyclotron, is used to treat a tumor region located at 30 cm. For this energy, the Bragg peak has to occur at the same distance of the tumor and with energy variation, the user must produce a several Bragg peaks through an area of about 5 × 3 mm2, which is the size of the tumor region.
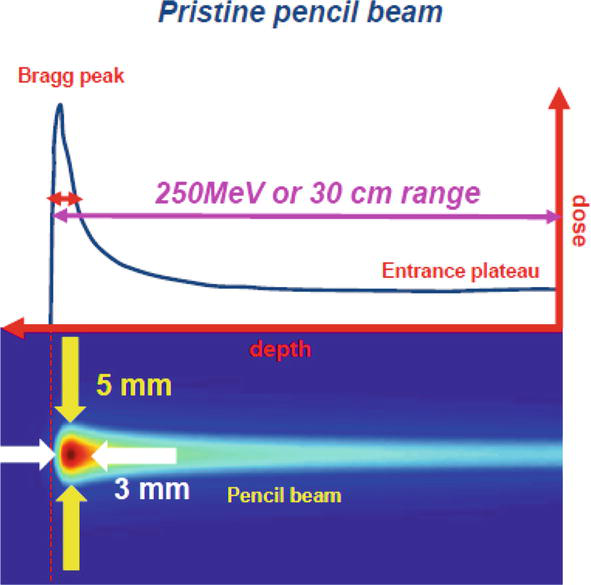
Figure 7.
Pencil beam scanning technique.
6. Accelerators used in the proton therapy
The protons beam used in radiation therapy is provided by two particle accelerators, cyclotrons, and synchrotrons, which produce proton beams at typical energies between 70 and 250 MeV [12]. The cyclotron, mainly used for proton therapy, is a machine which delivers protons with fixed energy. In order to adjust the energy to a level suitable for the treatment depth, the emitted proton beam will be filtered in an energy selection system. Whereas, in the synchrotrons, mainly used for carbon ions, the energy of proton beam can be controlled, and no energy degradation system is required. Therefore, proton beams with narrower Bragg peaks are produced by the synchrotron. Compared to the cyclotron, the generated spot sizes are potentially smaller.
7. Biological aspects of PBT
PBT is still considered as sparsely ionizing irradiation, which has a similar biological effect as photons. The biological aspect in radiotherapy is characterized by the relative biological effectiveness (RBE) which is a conceptual constant determined from experimental data. We also define the biological effective dose or relative biological effectiveness (RBE) weighted, which is the product of the physical dose with the relative biological effectiveness (RBE) factor [13]. The biological dose is expressed as Gy RBE. In clinical practice for the proton therapy, to get the biological effective dose, the physical dose of protons must be multiplied with a fixed relative biological effectiveness (RBE) factor of 1.1. The RBE of protons is defined as the dose of a reference radiation divided by the proton dose to achieve the same biological effect. The proton therapy treatments in almost all institutions worldwide are based on a single value of RBE of 1.1. However, there is growing debate over whether this fixed RBE of 1.1 is still appropriate for protons. In this way, preclinical data resulting from frequent physical and biological experiments stated that there is strong indication that the RBE factor of protons may be variable [14]. In this way, considerable efforts have been made by the international scientific community during the last decades to gain a quantitative estimate on the relative biological effectiveness of proton radiation, yet the question remains open and is a matter of controversy. This uncertainty is supported by the significant differences between reported measured RBE values and those predicted by available biological effect models [13, 15]. Moreover, biological experiments have evidenced RBE dependencies on dose level, linear energy transfer (LET), and tissue type.
In a similar way, it was reported that the value of 1.1 for relative biological effectiveness entails an uncertainty in the determination of the biological dose which is largest at most distal part of the proton track [16, 17]. In two lung cancer cell lines, H460 and H1437, irradiated with protons, the experimental results revealed that the measured RBE profiles strongly depend on the physical characteristics such as dose-mean lineal energy yd [18]. On the other hand, Harrabi et al. [19] reported that the increased incidence of asymptomatic radiation-induced brain injuries with an increased linear energy transfer (LET) average, observed in this cohort, especially in the distal part of the spread-out Bragg peak, provides strong clinical evidence to support the hypothesis that the relative biological effectiveness of protons is variable and different to the fixed factor of 1.1. Additionally, a thorough RBE review presented average values of 1.1, 1.15, 1.35, and 1.7 at the entrance, center, distal edge, and distal fall-off of the spread-out Bragg peak, respectively [14], indicating the increased RBE toward the distal edge of the treatment field.
In Figure 8, the physical dose, the biological dose (RBE-weighted dose), and the linear energy transfer (LET) evolution are represented with respect to penetration depth of proton beam of 220 MeV [20]. This figure summarizes all the results cited above. Firstly, we can identify the tumor area extended from a depth of 20–30 cm, which is also the region of spread-out Bragg peak [SOBP] plateau. The RBE, which represents the ratio (biological dose / the physical dose), remains constant at the entrance, center, and distal edge of the Bragg curve, whereas, it increases in the tumor region [14]. Furthermore, as reported above [19], the RBE increases with increasing linear energy transfer (LET). These interpretations are well schematized in Figure 9.
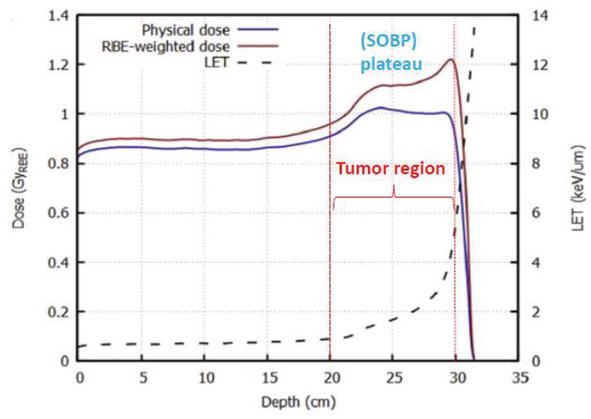
Figure 8.
Physical dose, biological dose (RBE- weighted dose), and the linear energy transfer (LET) evolution versus penetration depth of proton beam of 220 MeV.
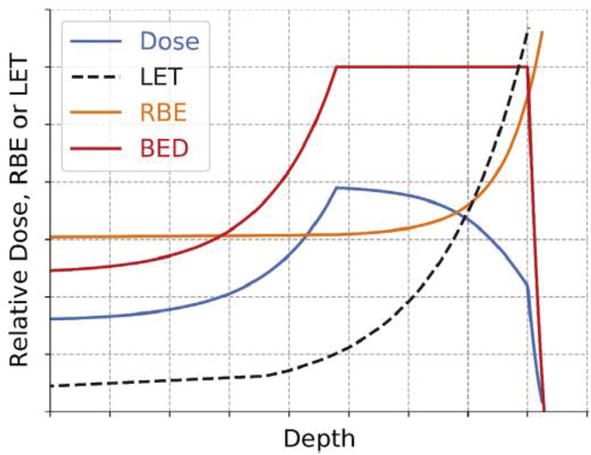
Figure 9.
Schematic diagram of designed dose profile (blue), linear energy transfer (LET; black), relative biological effectiveness (RBE; orange), and biological effective dose (BED; red) with depth [
Further investigations will be required to evaluate the quantitative effects of RBE variation on treatment planning and tumor control and toxicity to normal tissues. A better understanding of RBE variability would enable the development of safer and more effective proton treatments.
8. Clinical evidence
Due to its physical and biological characteristics, the PBT can be used in several treatment of the tumor in several parts and organs in the body. Previous studies [13, 14] suggested that patients with head and neck cancer may benefit from PBT. For patients with chest tumors, PBT may be an effective and safe treatment option. In comparison to photons, PBT plans may deliver lower doses to the adjacent organs at risk, such as the esophagus, lungs, and bone marrow, thus improving the therapeutic ratio [21]. In addition, it has been demonstrated that PBT is a safe and effective method for patients with abdominal and pelvic tumors especially localized prostate cancer [16, 17]. Moreover, among the options offered by radiotherapy, PBT is relevant choice to reduce unnecessary irradiation dose in pediatric patients. For this reason, PBT may be useful for the treatment of pediatric cancer [22]. PBT has also a potential benefit in reducing the irradiated dose to normal brain tissue to prevent cognitive dysfunction. Additionally, dose escalation might be possible in radioresistant brain tumors such as high-grade gliomas. PBT may allow dose intensification of chemotherapy by improved hematological tolerance in gastrointestinal, thoracic, and other types of cancer [23].
9. Future advancement of proton therapy
Proton therapy has evolved, and future predictions include smaller systems, more sophisticated proton dosimetry, and devices that manipulate the proton beam. The main challenges of proton therapy are continued miniaturization of proton therapy technology, improved precision of beam delivery, enhanced proton dosimetry computation, and further shaping of the proton beam.
10. Conclusion
Proton beam therapy is the latest kind of radiation therapy, which exerts satisfactory curative effect. In recent years, an increasing number of patients have been treated with PBT worldwide. PBT can achieve a dose distribution that is generally superior to conventional external photon beam radiation. Compared to conventional radiotherapy, PBT proton therapy presents several benefits: Lower risk of radiation damage to tissues, higher radiation dose to the tumor, better likelihood that all tumor cells are destroyed. However, future studies must integrate, evaluate, and manage information associated with PBT, in order to provide patients with the optimal treatment while reducing injury to normal tissues and treatment costs, and to clearly determine which patients may benefit the most from PBT. The relative biological effect of PBT requires further investigation.
References
- 1.
Gaito S, Aznar MC, Burnet NG, Crellin A, France A, Indelicato D, et al. Assessing Equity of Access to Proton Beam Therapy: A Literature Review. Clinical Oncology. 2023; 35 :e528ee536. DOI: 10.1016/j.clon.2023.05.014 - 2.
Bloch F. Zur bremsung rasch bewegter teilchen beim durchgang durch materie. Annalen der Physik. 1933; 408 (3):285-320. DOI: 10.1002/andp.19334080303 - 3.
Bortfeld T. An analytical approximation of the bragg curve for therapeutic proton beams. Medical Physics. 1997; 24 (12):2024-2033. DOI: 10.1118/1.598116 - 4.
Wilson RR. Radiological use of fast protons. Radiology. 1946; 47 :487-491. DOI: 10.1148/47.5.487 - 5.
Paganetti H, Niemierko A, Ancukiewicz M, Gerweck LE, Goitein M, Loeffler JS, et al. Relative biological effectiveness (RBE) values for proton beam therapy. International Journal of Radiation Oncology, Biology, Physics. 2002; 53 :407-421. DOI: 10.1016/s0360-3016(02)02754-2 - 6.
Wilkens JJ, Oelfke U. Direct comparison of biologically optimized spread-out Bragg peaks for protons and carbon ions. International Journal of Radiation Oncology, Biology, Physics. 2008; 70 (1):262-266. DOI: 10.1016/j.ijrobp.2007.08.029 - 7.
Jette D, Chen W. Creating a spread-out Bragg peak in proton beams. Physics in Medicine and Biology. 2011; 56 (11):N131-N138. DOI: 10.1088/0031-9155/56/11/N01 - 8.
Rezaee L. Design of spread-out Bragg peaks in hadron therapy with oxygen ions. Report Practical Oncology Radiotherapy. 2018; 23 (5):433-441. DOI: 10.1016/j. rpor.2018.08.004 - 9.
Schardt D, Elsässer T, Schulz-Ertner D. Heavy-ion tumor therapy: Physical and radiobiological benefits. Reviews of Modern Physics. 2010; 82 :383-425. DOI: 10.1103/RevModPhys.82.383 - 10.
Farr JB, Moyers MF, Allgower CE, Bues M, Hsi W-C, Jin H, et al. Clinical commissioning of intensity-modulated proton therapy systems: Report of AAPM Task Group 185. Medical Physics. 2021; 48 :e1-e30. DOI: 10.1002/mp.14546 - 11.
Moyers MF, et al. Physical Uncertainties in the Planning and Delivery of Light Ion Beam Treatments (Report of the AAPM Task Group 2020). Alexandria, VA: American Association of Physicists in Medicine AAPM; 2020. DOI: 10.37206/200 - 12.
Hagan WK, Colborn BL, Armstrong TW, Allen M. Radiation shielding calculations for a 70- to 250-MeV proton therapy facility. Nuclear Science Engineering. 1988; 98 :272-278. DOI: 10.13182/NSE88-A22328 - 13.
Mohan R, Grosshans D. Proton therapy – Present and future. Advanced Drug Delivery Reviews. 2017; 109 :26-44. DOI: 10.1016/j.addr.2016.11.006 - 14.
Paganetti H. Relative biological effectiveness (RBE) values for proton beam therapy. Variations as a function of biological endpoint, dose, and linear energy transfer. Physics in Medicine and Biology. 2014; 59 :R419-R472. DOI: 10.1088/0031-9155/59/22/R419 - 15.
Polster L, Schuemann J, Rinaldi I, Burigo L, McNamara AL, Stewart RD, et al. Extension of TOPAS for the simulation of proton radiation effects considering molecular and cellular endpoints. Physics in Medicine and Biology. 2015; 60 :5053. DOI: 10.1088/0031-9155/60/13/5053 - 16.
Lühr A, von Neubeck C, Pawelke J, Seidlitz A, Peitzsch C, Bentzen SM, et al. Radiobiology of proton therapy: Results of an international expert workshop. Radiotherapy and Oncology. 2018; 128 :56-67. DOI: 10.1016/j.radonc.2018.05.018 - 17.
Sørensen BS, Pawelke J, Bauer J, Burnet NG, Dasu A, Høyer M, et al. Does the uncertainty in relative biological effectiveness affect patient treatment in proton therapy? Radiotherapy and Oncology. 2021: 163 :177-184. DOI: 10.1016/j.radonc.2021.08.016 - 18.
Bronk L, Guan F, Patel D, Ma D, Kroger B, Wang X, et al. Mapping the relative biological effectiveness of proton, helium and carbon ions with high-throughput techniques. Cancers (Basel). 2020; 12 (12):3658. DOI: 10.3390/cancers12123658 - 19.
Harrabi SB, von Nettelbladt B, Gudden C, Adeberg S, Seidensaal K, Bauer J, et al. Radiation induced contrast enhancement after proton beam therapy in patients with low grade glioma – How safe are protons? Radiotherapy and Oncology. 2022; 167 :211-218. DOI: 10.1016/j.radonc.2021.12.035 - 20.
Jones B, McMahon SJ, Prise KM. The radiobiology of proton therapy: Challenges and opportunities around relative biological effectiveness. Clinical Oncology. 2018:1-8. DOI: 10.1016/j.clon.2018.01.010 - 21.
Berman AT, James SS, Rengan R. Proton beam therapy for non-small cell lung cancer: Current clinical evidence and future directions. Cancers (Basel). 2015; 7 :1178-1190. DOI: 10.3390/cancers7030831 - 22.
Ahmed KA, Demetriou SK, McDonald M, Johnstone PA. Clinical benefits of proton beam therapy for tumors of the skull base. Cancer Control. 2016; 23 :213-219. DOI: 10.21037/cco.2016.07.05 - 23.
Sejpal S, Komaki R, Tsao A, Chang JY, Liao Z, Wei X, et al. Early findings on toxicity of proton beam therapy with concurrent chemotherapy for non-small cell lung cancer. Cancer. 2011; 117 :3004-3013. DOI: 10.1002/cncr.25848