Abstract
This chapter aims to briefly review the main theoretical and finite element simulation results on the elastic properties and yield strengths of regular hexagonal honeycombs, Kelvin open cell foams, random irregular Voronoi honeycombs and open-cell foams, and discuss about their deformations mechanisms. The book chapter further introduces the effects of other parameters such as the degree of cell regularity, imperfection of defects, cell size, solid volume fraction and material distribution, on the elastic properties and yield strengths of these cellular materials. Voronoi honeycombs and open cell foams can be related to their mechanical properties.
Keywords
- honeycombs
- open-cell foams
- elastic properties
- yield strengths
- size effects
1. Introduction
Living natural materials are usually cellular materials, examples of which include bones and wood. Such structures tend to use as little as possible solid material to provide sufficient mechanical properties (e.g., strength, stiffness, and energy absorption capacity) and the desired physical or biological functions. In general, open-celled cellular materials can be classified into two types. The first type is honeycombs which are often treated as 2D materials in some studies, and the other type is open cell foams which are 3D materials and are not suitable to be treated as 2D materials.
In practical applications, there are many different types of regular or irregular honeycombs, open-cell foams and two-dimensional (2D) or three-dimensional (3D) auxetic cellular materials. Numerous publications are relevant to elastic buckling, plastic collapse and dynamic performance, which are involved in geometric nonlinearity, material nonlinearity and strain rate effects. In most engineering applications, the strength and stiffness are the most important mechanical properties of cellular materials, which are essential to ensure the structural integrity and to enable the relevant physical functions. Due to the page limit, this chapter only briefly summarizes the key theoretical and simulation research results on the static strength and stiffness of low-density regular hexagonal honeycombs, Kelvin open-cell foams and their random irregular Voronoi structures, and then briefly introduces the effects of some key factors on these properties. Sections 2 and 3 present the elastic properties for the first type of open-cell cellular materials, i.e. honeycombs; Sections 4 and 5 present the these properties for open-celled foams.
The honeycomb and open-cell foam structures could be used under different environmental conditions such as varying and extreme temperature and humidity. In these cases, as long as the honeycombs and open-cell foams are initially sufficiently strong and stiff, their long-term structural integrity and mechanical performance mainly depend on the solid material from which the honeycombs and the open-cell foams are made.
2. The elastic properties of honeycombs
There are different types of regular honeycombs, for example, triangular honeycombs and square honeycombs. In most relevant research works, honeycombs are treated as 2D materials. To partition a two-dimensional domain into a cellular structure with
where
Regular hexagonal honeycombs at different size scales have five independent elastic constants and the analytical results of all these elastic constants are obtained [4]. It has been found that when the cell wall thickness is small, these properties are size-dependent due to the strain gradient effects at the micro-meter scale and the surface effects at the nanometer scale [4]. In general, the smaller the cell size, the stiffer the honeycombs. When the cell wall thickness is at the millimeter scale or larger, the elastic constants of regular hexagonal honeycombs depend only on
Silva et al [5] used finite element method to simulate the elastic properties of non-periodic Voronoi honeycombs. Zhu et al., for the first time, defined the degree of cell regularity for random irregular Voronoi honeycombs, and studied the effects of cell regularity on the elastic properties of periodic Voronoi honeycombs [6] and related their geometric properties of the irregular Voronoi cells [7] to the elastic properties of irregular honeycombs. To partition an area
To partition the same area into
When
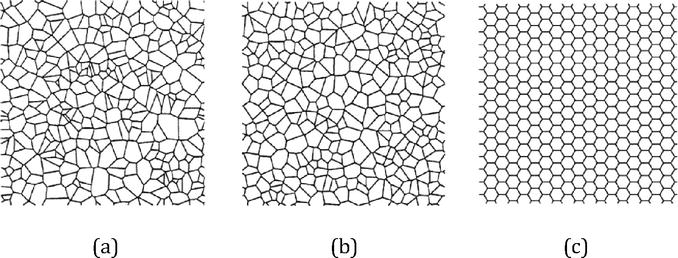
Figure 1.
Periodic honeycombs with 300 complete cells [
Zhu et al. [6] used finite element method (ABAQUS Timoshenko beam elements) and periodic random representative volume element (RVE) models, as shown in Figure 1, to study the effects of relative density and cell regularity on the in-plane elastic properties (i.e., Young’s modulus, shear modulus, Poisson’s ratio, and bulk modulus) of low-density random Voronoi honeycombs. They found that the elastic properties are in-plane isotropic. The obtained effects of cell regularity on the in-plane Young’s modulus and bulk modulus of Voronoi honeycombs with
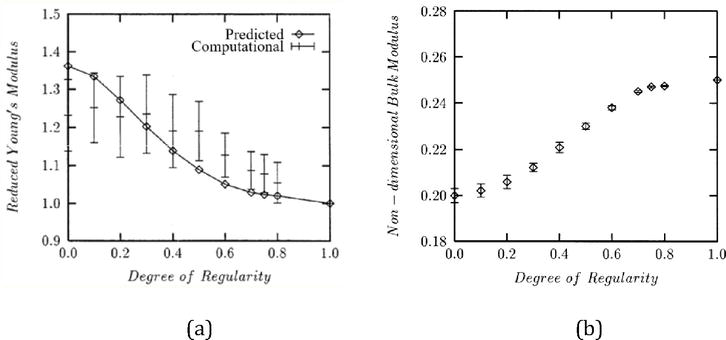
Figure 2.
Effects of cell irregularity on the in-plane Young’s modulus (a) and bulk modulus (b) of random irregular Voronoi honeycombs with
It is noted that due to the effects of axial compression and transverse shear of the cell walls, the normalized Young’s moduli and shear moduli of both regular hexagonal honeycombs and Voronoi honeycombs decrease with the increase of the relative density. This is because compared to the bending deformation of the cell walls, the deformations caused by the axial compression and transverse shear are very small and negligible when the honeycomb relative density is very small. However, they increase with the honeycomb relative density and could become comparable if the relative density is large, making the normalized Young’s moduli and shear moduli smaller with the increase of the honeycomb relative density. Due to the strain gradient effect at the micro-scale and the surface effect at the nano-scale, the thinner the cell walls, the larger the in-plane Young’s modulus of the regular or random irregular Voronoi honeycombs [4, 8]. For single-level nano-sized honeycombs or multi-level nano-structured hierarchical honeycombs, both their elastic properties and geometric properties could be controlled to vary over large ranges by applying an electric potential to change the surface stress of the nano-sized cell walls [4, 8]. At the micro- or macro-scale, the out-of-plane elastic constants of both regular hexagonal and random irregular Voronoi honeycombs with a uniform cell wall thickness are
For the in-plane uniaxial deformation of low-density regular and irregular honeycombs at different size scales, cell wall bending is always the dominant deformation mechanism. When a honeycomb undergoes in-plane deformation, the largest bending moment usually occurs at the cell wall junction. Thus, material distribution along the cell wall can significantly affect the in-plane Young’s modulus of honeycombs. By properly increasing solid material along the cell wall from the middle to the junction, the in-plane Young’s modulus can be remarkably increased [9, 10]. In addition, imperfections such as inclusions and holes can sharply reduce the in-plane Young’s modulus of honeycombs [11].
3. The yield strength of honeycombs
Many people [3, 10, 11, 12, 13, 14] have studied the yield strength of regular hexagonal and random Voronoi honeycombs. For a low-density regular hexagonal honeycomb with a uniform cell wall thickness and a small ratio of
If the same low-density regular hexagonal honeycomb is made of an elastic and perfectly plastic material, its in-plane full yield strength is 50% higher than the initial yield strength and given as [3].
In Eqs. (4) and (5),
For a low-density regular hexagonal honeycomb with a uniform cell wall thickness and a large ratio of
If the same low-density regular hexagonal honeycomb is made of an elastic and perfectly plastic material, the full yield strength can be obtained as [10].
The in-plane initial or full shear yield strength of a low-density regular honeycomb can be obtained by dividing the corresponding in-plane initial or full uniaxial yield strength by
When a regular hexagonal honeycomb made of an elastic and perfectly plastic material is under out-of-plane uniaxial tension or compression (i.e., under uniaxial tension or compression in direction 3), its initial plastic yield strength is the same as the full yield strength, and is given as
It is noted that the results on the in-plane and out-of-plane elastic buckling, plastic collapse and dynamic behaviors are not included here due to the page limit.
4. The elastic properties of open-cell foams
Open cell foams are another type of cellular materials, and their typical geometric structure can be seen in Figure 3(a) [15]. Taking strut bending as the sole deformation mechanism and using dimensional analysis, Gibson and Ashby [3, 18] have obtained the Young’s modulus and shear modulus for low-density three-dimensional open-cell foams and their results are given as
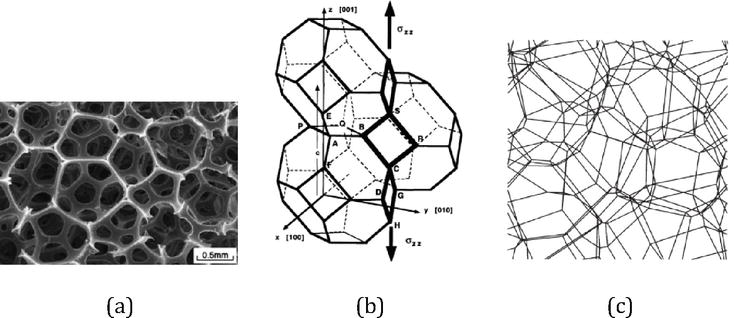
Figure 3.
Geometric structure and models of three-dimensional open-cell foams, (a) the actual structure of an open cell foam [
In Eq. (8),
Periodic unit cell structures or periodic representative volume elements (RVEs) are often used to study the effective mechanical properties of cellular materials. Taking strut bending, torsion and axial stretching/compression as the deformation mechanisms, Zhu et al. [16] have used the tetrakaidecahedron (also called BCC or Kelvin foam) structural model, as shown in Figure 3(b), to analyze the elastic properties of low-density open-cell foams, obtained the general expressions of the Young’s modulus
Where the foam relative density
The theoretical Young’s modulus given by Eq. (9) is exactly the same as Gibson and Ashby’s experimental result
Thus, the elastic properties of the low-density tetrakaidecahedron (or BCC or Kelvin) open-cell foams are almost isotropic (i.e.,
A real open-cell foam usually has a random irregular geometric structure. Thus, a 3D random Voronoi representative volume element (RVE) can more realistically reflect the geometric structure and the mechanical properties of open-cell foams. Zhu et al., for the first time, defined the degree of regularity for random irregular Voronoi open-cell foams, and studied the effects of cell regularity on the elastic properties of periodic Voronoi open-cell foams [17] and related their geometric properties [19] to their elastic properties. To partition a space
To construct a random irregular Voronoi tessellation with
For a regular lattice with identical tetrakaidecahedral cells,
Zhu et al. [17] developed a computer code to construct periodic 3D random irregular Voronoi open-cell foams as shown in Figure 3(c), and then used ABAQUS Timoshenko beam elements and periodic boundary conditions to study the effects of relative density and cell regularity on the Young’s modulus, shear modulus, Poisson’s ratio and bulk modulus of Voronoi open-cell foams. Figure 4 shows the effects of cell regularity on the Young’s modulus and bulk modulus of low-density open-cell foams with
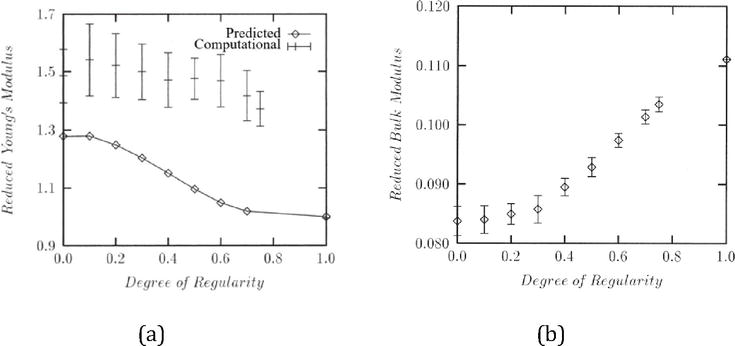
Figure 4.
Effects of cell regularity on the (a) Young’s modulus and (b) bulk modulus of random Voronoi open-cell foams with
Zhu et al. [17] also developed a springs-in-parallel model to relate the geometric properties [19] to the elastic properties of 3D random irregular Voronoi open-cell foams, and to qualitatively predict how cell regularity affects the Young’s modulus of the random irregular Voronoi open-cell foams, as shown by the predicted results in Figure 4(a). Although the springs-in-parallel model could well predict the trend of the cell regularity effects, the predicted Young’s moduli exhibit large deviations from the exact results obtained from the finite element simulations. This model is just an attempt to qualitatively explain the reason why an irregular open-cell foam has a larger Young’s modulus than a more regular open-cell foam. So far, there is no available analytical model that could accurately predict the effects of cell regularity on the elastic properties of irregular open-cell foams.
For low-density open-cell foams with
5. The yield strength of open-cell foams
Many people [3, 18, 22, 23, 25, 28, 29, 30] have studied the plastic collapse strength of different types of open-cell foams. For low-density open-cell foams, the plastic collapse strength is given by [3, 18].
or
As strut bending and torsion are the dominant deformation mechanisms and the largest bending moment usually occurs at the strut junctions, allocating more solid material near the strut junctions could increase the yield strength [22, 23]. In addition, defects could significantly reduce the yield strength of open-cell foams [25]. It is found that low-density random irregular Voronoi open-cell foams are stiffer and stronger than Kelvin open-cell foams for compressive strain up to 0.15 [31] and have a larger yield strength than Kelvin open-cell foams [22]. In addition, it has been experimentally found that for Au open-cell foams with the same relative density and the size of the cell struts at the nanometer scale, the smaller the cell struts, the larger the yield strength of the nano open-cell foams [32].
6. Conclusions
There are thousands of publications on the mechanical properties of honeycombs and open-cell foams. Theoretical analyzes are usually based on specific geometric models, and many different types of geometric models have been proposed and analyzed for honeycombs and open-cell foams. Regular hexagonal honeycomb, which has five independent elastic constants, is the best structural model for regular honeycombs [1, 3], and regular Kelvin foam, which has three independent elastic constants, is the best structural model for regular open-cell foams [16]. Natural honeycombs and open-cell foams, however, always have a certain degree of irregularity. Thus, random irregular 2D [6, 7] and 3D [17, 19, 31] Voronoi tessellations with different degrees of cell regularity are the most ideal geometric models for the analyzes of the mechanical properties of honeycombs and open-cell foams.
Due to the page limit, this chapter just briefly reviews/introduces the generally recognized knowledge about the elastic properties and yield strength of honeycombs and open-cell foams. The mechanical properties of both honeycombs and open-cell foams strongly depend on their relative density, and the larger the relative density, the stiffer and stronger the honeycombs and open-cell foams. In addition to the effects of relative density, their Young’s modulus, shear modulus and yield strength are proportional to those of the solid material from which the honeycombs or open-cell foams are made. It has been found that defects could significantly reduce the strength and stiffness of honeycombs [11, 12] and open-cell foams [25].
For the in-plane deformation of low-density honeycombs, cell wall bending is the dominant deformation mechanism, although the axial compression and transverse shear of the cell walls also play an important role [1, 2, 3, 4, 5, 6]. Low-density random irregular honeycombs have larger in-plane Young’s modulus, smaller in-plane yield strength and bulk modulus, and the same out-of-plane Young’s modulus and yield strength compared to the same density regular hexagonal honeycombs [6, 12]. For low-density open-cell foams, strut bending and torsion are the dominant deformation mechanisms, although the axial compression and transverse shear of the cell struts also play an important role [16, 17, 31]. In general, random irregular open-cell foams have larger Young’s modulus and yield strength, and smaller bulk modulus than those of the same density regular Kelvin open-cell foam [16, 17, 31].
As the largest bending moment usually occurs at the cell wall or cell strut junctions, allocating more solid material near the cell wall or cell strut junctions could significantly increase the stiffness and yield strength of honeycombs and open-cell foams [9, 10, 12, 22, 23, 24]. Due to the strain gradient effects at the micro-scale and the surface effects at the nanometer scale, the thinner the cell walls or cell struts, the stiffer and stronger the honeycombs or open-cell foams with the same relative density [4, 8, 26, 27, 32]. For nano-sized single-level or nano-structured multi-level hierarchical honeycombs and open-cell foams, their mechanical and geometric properties could be controlled to vary over a large range by application of an electric potential [4, 8, 26, 27].
References
- 1.
Gibson LJ, Ashby MF, Schajer GS, Robertson CI. The mechanics of two-dimensional cellular materials. Proceeding of the Royal Society of London. 1982; 382 :25-42 - 2.
Warren WE, Kraynik AM. Foam mechanics: The linear elastic response of two-dimensional spatially periodic cellular materials. Mechanics of Materials. 1987; 6 :27-37 - 3.
Gibson LJ, Ashby MF. Cellular Solids: Structure and Properties. 2nd ed. Cambridge: Cambridge University Press; 1997 - 4.
Zhu HX. Size-dependent elastic properties of micro- and nano-honeycombs. Journal of the Mechanics and Physics of Solids. 2010; 58 :696-709 - 5.
Silva MJ, Hayes WC, Gibson LJ. The effects of non-periodic microstructure on the elastic properties of two-dimensional cellular solids. International Journal of Mechanical Sciences. 1995; 37 :1161-1177 - 6.
Zhu HX, Hobdell JR, Windle AH. Effects of cell irregularity on the elastic properties of 2D Voronoi honeycombs. Journal of the Mechanics and Physics of Solids. 2001; 49 :857-870 - 7.
Zhu HX, Thorpe SM, Windle AH. The geometrical properties of irregular 2D Voronoi tessellations. Philosophical Magazine A. 2001; 81 :2765-2783 - 8.
Zhu HX, Zhang HC, You JF, Kennedy D, Wang ZB, Fan TX, et al. The elastic and geometrical properties of micro- and nano-structured hierarchical random irregular honeycombs. Journal of Materials Science. 2014; 49 :5690-5702 - 9.
Li K, Gao XL, Subhash G. Effects of cell shape and cell wall thickness variations on the elastic properties of two-dimensional cellular solids. International Journal of Solids and Structures. 2005; 42 :1777-1795 - 10.
Zhu HX, Chen CY. Combined effects of relative density and material distribution on the mechanical properties of metallic honeycombs. Mechanics of Materials. 2011; 43 :276-286 - 11.
Chen C, Lu TJ, Fleck NA. Effect of inclusions and holes on the stiffness and strength of honeycombs. International Journal of Mechanical Sciences. 2001; V43 :487-504 - 12.
Chen C, Lu TJ, Fleck NA. Effect of imperfections on the yielding of two-dimensional foams. Journal of the Mechanics and Physics of Solids. 1999; 47 :2235-2272 - 13.
Zhu HX. Large deformation pure bending of an elastic plastic power-law-hardening wide plate: Analysis and application. International Journal of Mechanical Sciences. 2007; 49 :500-514 - 14.
Silva MJ, Gibson LJ. The effects of non-periodic microstructure and defects on the compressive strength of two-dimensional cellular solids. International Journal of Mechanical Sciences. 1997; 39 :549-563 - 15.
Gao G, Qi M, Li Y. Random equilateral Kelvin open-cell foam microstructures: Cross-section shapes, compressive behavior, and isotropic characteristics. Journal of Cellular Plastics. 2018; 54 :53-72 - 16.
Zhu HX, Knott JF, Mills NJ. Analysis of the elastic properties of open-cell foams with tetrakaidecahedral cells. Journal of the Mechanics and Physics of Solids. 1997; 45 :319-343 - 17.
Zhu HX, Hobdell JR, Windle AH. The effects of cell irregularity on the elastic properties of open cell foams. Acta Materialia. 2000; 48 :4893-4900 - 18.
Gibson LJ, Ashby MF. The mechanics of three-dimensional cellular materials. Proceedings of the Royal Society A: Mathematical, Physical and Engineering Sciences. 1982; 382 :43-49 - 19.
Zhu HX, Zhang P, Balint D, Thorpe SM, Windle AH, Lin J. The effects of regularity on the geometrical properties of Voronoi tessellations. Physica A. 2014; 406 :42-58 - 20.
Christenson RM. Mechanics of low density materials. Journal of the Mechanics and Physics of Solids. 1986; 34 :563-578 - 21.
Warren WE, Kraynik AM. The linear elastic properties of open-cell foams. Journal of Applied Mechanics. 1988; 55 :341-346 - 22.
Mills NJ. The high strain mechanical response of the wet Kelvin model for open-cell foams. International Journal of Solids and Structures. 2007; 44 :51-65 - 23.
Jang WY, Kyriakides S, Kraynik AM. On the compressive strength of open-cell metal foams with Kelvin and random cell structures. International Journal of Solids and Structures. 2010; 47 :2872-2883 - 24.
Zargarian A, Esfahanian M, Kadkhodapour J, Ziaei-Ra S. Effect of solid distribution on elastic properties of open-cell cellular solids using numerical and experimental methods. Journal of the Mechanical Behavior of Biomedical Materials. 2014; 37 :264-273 - 25.
Lu ZX, Liu Q, Huang JX. Analysis of defects on the compressive behaviors of open-cell foams. Materials Science and Engineering A. 2011; 530 :285-296 - 26.
Zhu HX. Size-dependent elastic properties of micro- and nano-open-celled foams. Structural Longevity. 2011; 5 :17-24 - 27.
Zhu HX, Wang ZB. Size-dependent and tunable elastic and geometric properties of hierarchical nano-porous materials. Science of Advanced Materials. 2013; 5 :677-686 - 28.
Deshpande VS, Fleck NA. Isotropic constitutive models for metallic foams. Journal of the Mechanics and Physics of Solids. 2000; 48 :1253-1283 - 29.
Amsterdam E, De Hosson JTM, Onck PR. On the plastic collapse stress of open-cell aluminum foam. Scripta Materilia. 2008; 59 :653-656 - 30.
Karamooz Ravari MR, Kadkhodaei M, Badrossamay M, Rezaei R. Numerical investigation on mechanical properties of cellular lattice structures fabricated by fused deposition modeling. International Journal of Mechanical Sciences. 2014; 88 :154-161 - 31.
Zhu HX, Windle AH. Effects of cell irregularity on the high strain compression of open-cell foams. Acta Materialia. 2002; 50 :1041-1052 - 32.
Hodge AM, Biener J, Hayes JR, Bythrow PM, Volkert CA, Hamza AV. Scaling equation for yield strength of nanoporous open-cell foams. Acta Materilia. 2007; 55 :1343-1349