Pros and cons of zebrafish as a model system compared with other species.
Abstract
Assessing the welfare of aquatic ecosystems has gained immense importance within the scientific community, elevating ecotoxicology to a fundamental discipline. This field utilizes bioindicators, often referred to as model organisms, to investigate the harmful effects of environmental pollution. Amid this array of biological markers, the zebrafish stands out as an exceptional contender. The primary objective of this present investigation is to highlight the complete information available in the literature about the utilization of zebrafish in ecotoxicology. Zebrafish are commonly utilized in research to study reproductive and embryonic toxicity due to their intricate sex-determination mechanism, which is significantly impacted by environmental factors. Similarly, this particular fish species is also employed in studying neuronal signaling, behavior, and DNA damage from pollutants due to their susceptibility and swimming abilities around day five post-fertilization. In addition to the established methodologies, recent advancements in environmental toxicology have incorporated zebrafish into emerging genome editing techniques. These innovative approaches provide fresh and compelling avenues for exploring detoxification mechanisms and organisms’ intricate adaptive responses to environmental stressors. In conclusion, zebrafish are important as model organisms, providing profound insights into diverse facets of ecotoxicological investigations. Furthermore, their relevance is further underscored by their adaptability to evolving genome editing technologies.
Keywords
- zebrafish
- ecotoxicology
- aquatic toxicology
- model organism
- bioindicator
1. Introduction
The proliferation of anthropogenic activities, such as industrialization, urbanization, and agriculture, has led to the release of diverse pollutants into aquatic ecosystems, including heavy metals, pesticides, pharmaceuticals, and organic compounds [1]. These pollutants disrupt the natural equilibrium of ecosystems, leading to impaired water quality, loss of biodiversity, and potential bioaccumulation of toxic substances throughout food chains [2]. Consequently, the need to assess the health of aquatic environments has become a pressing issue. Ecotoxicology serves as a crucial discipline in addressing the adverse effects of environmental pollution on aquatic organisms [3]. By systematically evaluating the toxicity, distribution, and persistence of contaminants in ecosystems, ecotoxicological studies provide essential insights into the potential risks posed by pollutants. These insights inform policy decisions, guide regulatory frameworks, and aid in developing effective pollution mitigation strategies.
Bioindicators, alternatively called ecological indicators or biological markers, are living organisms that exhibit heightened sensitivity to environmental shifts primarily induced by human activities [4]. This diverse spectrum of organisms, encompassing avian and amphibian species, as well as insects and fish, is pivotal as indicators of the overall well-being of ecosystems. Among this varied assemblage of bioindicators, the zebrafish emerges as a particularly noteworthy candidate, owing to its distinctive characteristics and genetic resemblance to humans [5]. As an illustration, the transparency of zebrafish embryos permits direct observation of developmental processes, facilitating the detection of abnormalities resulting from environmental stressors [6]. Moreover, their swift reproductive cycles enable the examination of multi-generational impacts, unveiling chronic exposure effects [7]. This chapter aims to highlight the importance of zebrafish as a model organism in ecotoxicology.
2. Zebrafish
2.1 Zebrafish as model organism
2.1.1 Phylogeny
Zebrafish is classified within the kingdom
2.1.2 Morphology
2.1.3 Life cycle
In the presence of a male, adult female zebrafish exhibit a reproductive pattern wherein they can spawn every 2 to 3 days, resulting in the deposition of up to 200 eggs per week. This mating occurs in the morning, and light is the primary stimulus that triggers this behavior. Within 30 minutes after dawn, the female zebrafish can lay the eggs, which are fertilized by the male’s released sperm. After fertilization, immediate embryonic development commences. If the eggs remain unfertilized, their growth halts following the initial cell divisions, ultimately leading to death. The zebrafish life cycle encompasses four principal stages: embryo, larva, juvenile, and adult (Figure 1) [6].
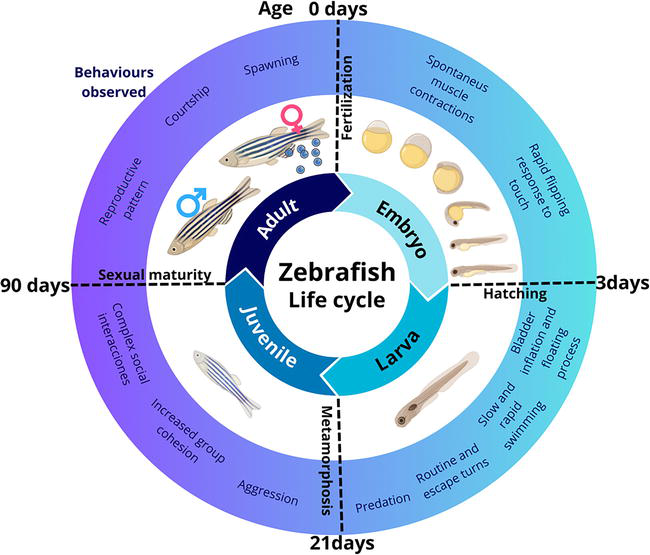
Figure 1.
Zebrafish life cycle: Embryo, larvae, juvenile, and adult.
Embryo:
Fertilized eggs (<0.7 mm) become transparent and develop rapidly [16]. Embryonic cells divide synchronously every 20 minutes [15]. Within the first 4 hours postfertilization (hpf), the fertilized egg transforms from a single cell into 1000 cells. Organogenesis (cell division and differentiation) occurs between 15 and 24 hpf. At 15.5 hpf, the tail bud becomes more prominent. Pigment formation starts in the retinal pigmented epithelial cells and dorsolateral melanophores in the skin by 28 hpf [17]. The heart begins to beat at approximately 30 hpf. Shortly after (36 hpf), all major organs and tissues formed within the transparent embryos [18]. By 37 hpf, the brain has nearly fully developed. The pancreas, liver, and bladder are recognizable at 49 hpf [19]. Eventually, the embryos sporadically hatch between 48 and 72 hpf.
Larva:
This stage extends from hatching to 21 days postfertilization (dpf) [20]. After hatching, the continuous anterodorsal protrusion of the mouth begins. Several days later, the swim bladder inflates with air, initiating the “floating “process [17]. The early larva starts swimming and moves its fins, jaw, and eyes. These actions promote respiration, the development of both rapid and slow movements, quick escape responses, food seeking, and predation on small prey [21].
Juvenile:
This stage is characterized by the zebrafish larvae undergoing metamorphosis (22 dpf), such as the complete loss of the larval fin fold. Juvenile characteristics are acquired, such as scales on the body and the formation of two rows of melanophores along the edge of each scale [6]. Furthermore, the pigment pattern on their skin becomes more pronounced during this process. The juvenile zebrafish possesses all the physical characteristics of an adult of its species except for sexual maturity. Social interactions among young fish become more complex, involving aggressive encounters and increased group cohesion [22].
Adults:
Approximately 90 days after fertilization, fish achieve sexual maturity and progress into reproductive adulthood [23]. Furthermore, within this temporal frame, discernible sexual dimorphism becomes apparent as males and females demonstrate the capacity to generate functional gametes and manifest secondary sexual attributes [24].
2.1.4 Advantages and constraints
The utilization of zebrafish as a biological model constitutes a profoundly invaluable asset within the realm of scientific investigation. This is attributed to its unique capacity to furnish insights across immediate and protracted in vivo research endeavors. Table 1 expounds upon the merits and demerits inherent in employing the zebrafish (
Aspect | Justification | Reference |
---|---|---|
Rapid Development | The zebrafish undergoes a brief three-day embryonic development and attains reproductive maturity within 3 months. This species demonstrates a remarkable capacity for prolific embryo production over a concise timeframe. | [6] |
Embryonic Transparency | The inherent transparency of zebrafish embryos enables the direct and noninvasive monitoring of intricate biological phenomena, such as the development of organs or the advancement of tumors. However, the opacity of tissues in other organisms constrains the extent of feasible observation. | [25] |
Genetic Manipulation | The comprehensive characterization of the zebrafish genome facilitates the manipulation of genetic elements, thereby providing a robust platform to investigate the precise roles of individual genes across diverse biological processes. | [26] |
Homology with Humans | Roughly 70% of the human gene repertoire possesses at least one discernible homolog in the zebrafish genome. Although precise gene-to-gene correspondence may not be uniform across the two species, many pivotal genetic pathways and fundamental processes exhibit conservation. This genetic analogy facilitates the elucidation of human biological mechanisms and the exploration of genetic afflictions and anomalies. | [27] |
Cost and Space Efficiency | The zebrafish presents itself as a miniature and easily manageable organism within laboratory settings, affording the advantage of diminished expenses and spatial prerequisites for its upkeep and experimental utilization, in stark contrast to mammalian models. This financial economy can manifest substantially, ranging from ratios of 1:100 to 1:1000 when compared against rodent counterparts. | [28] |
Fluorescent Labeling | Fluorescent markers possess the capability to designate proteins within zebrafish, facilitating noninvasive imaging and enabling the examination of intricate cellular and molecular mechanisms. | [29] |
Limited Biological Complexity | The zebrafish exhibits a comparatively reduced level of biological intricacy compared with organisms such as mice or rabbits. These latter animals possess biological systems more akin to human physiology, rendering them more suitable for conducting intricate investigations into complex diseases and physiological processes. | [30] |
Complex Behavior Studies Limitation | Zebrafish manifests comparatively less intricate behavioral patterns than highly evolved organisms such as mice or primates. This disparity in behavioral complexity imposes constraints on the comprehensive investigation of specific facets of animal behavior. | [31] |
Immune System Dissimilarity | The zebrafish’s immune system exhibits notable distinctions compared to that of high vertebrates. This dissimilarity could pose limitations for research into immune responses and exploring precise immune mechanisms, as the zebrafish possesses a less complex immune system. | [32] |
Tissue Size and Manipulation Restrictions | The small size of zebrafish and its tissues and organs could limit the execution of experiments requiring larger tissue quantities or more complex surgical procedures. | [33] |
Table 1.
2.2 Zebrafish in aquatic ecotoxicology
2.2.1 Reproductive and embryonic toxicity
Mammals exhibit dimorphic sex chromosomes and follow a male heterogametic system for sex determination [34]. In this system, a particular gene known as SRY (sex-determining region Y) plays a pivotal role by acting as a genetic switch to initiate the male developmental pathway within mammals’ bipotential gonads [35]. This means that the presence or absence of the SRY gene determines whether an individual develops as male or female during embryonic development. Unlike mammals, zebrafish do not possess a straightforward sex determination cascade. Instead, their sex-determination system is intricate and influenced by genetic and environmental factors. Research has shown that various elements, such as surrounding temperature [36], exposure to sex hormones such as estrogen and androgen [37], and oxygen availability [38] play significant roles in gonad ontogenetic differentiation in zebrafish. Endocrine-disrupting chemicals (EDCs) are external agents that profoundly impact the reproduction and development of organisms by interfering with endogenous hormones’ natural synthesis, release, metabolism, and functioning [39]. These chemical compounds have been identified in various environmental compartments, such as air, soil, water, wildlife, and human tissues [40]. Thus, EDCs’ effects on living organisms are of significant concern. Over the past few decades, investigations have concentrated on the adverse implications of EDCs on zebrafish after brief or prolonged exposure [41, 42, 43, 44, 45]. Most of these studies have targeted specific developmental stages in the zebrafish life cycle: adult, larvae, or embryo. In the broader context, it has been observed that adult zebrafish exhibit comparatively lower sensitivity to endocrine disruption when compared with developing embryos [46]. Nonetheless, this does not exempt them from experiencing detrimental effects on their gonads because of exposures to EDCs. Recent investigations have shed light on various consequences of such endocrine disruption in adult zebrafish. These effects include a reduced proportion of mature spermatozoa and spermatids [47]. Moreover, there is a decrease in the proportion of vitellogenin oocytes, coupled with an increased occurrence of atretic follicles, indicating a disruption in the normal ovarian follicle development process [48].
A clear difference between adult zebrafish and their embryonic and larval counterparts is evident in reproductive testing. Notably, during the early life stages, all zebrafish, regardless of gender, possess ovary-like gonads [46]. It is during the later stages of development that the definitive establishment of bisexual differentiation takes place [49]. In light of this information, recent research has suggested that the exposure of zebrafish to EDCs during sexual differentiation can lead to a skewed sex ratio, often resulting in an excess of female individuals [50, 51]. Although an initially inconspicuous skewed sex ratio might not manifest immediate detriment to the environment, it can potentially perturb the reproductive cycle of organisms and engender transgenerational effects. Transgenerational effects pertain to the potential negative consequences of exposures during early development that extend beyond the initial generation, leading to enduring repercussions on reproductive and developmental processes in subsequent generations. Several authors, for instance, have described that subjecting zebrafish to EDCs can lead to diverse effects, including elevated vitellogenin levels [51], feminization [52], and delayed production and development of their offspring [7, 53]. From the latter one, it is noteworthy to indicate that not only the transgenerational exposure nor the EDCs can delay or affect the embryonic development of zebrafish. Over the past decade, several authors have extensively documented the impact of various pharmaceutical and personal care products on zebrafish growth and development [54]. These studies have consistently revealed that exposure to these substances can significantly alter zebrafish’s normal developmental processes, ultimately leading to the prompt occurrence of malformations in these organisms [55]. For instance, a study carried out in 2022 demonstrated that zebrafish embryos exhibited scoliosis, pericardial edema, and yolk deformation following a 96-hour exposure to microplastics [56]. Herein, it is pertinent to highlight that the authors emphasize these deformations, and these deformations could arise from a reduction in the availability of oxygen and nutrients because of the accumulation of plastic particles on the surface of the embryos’ chorion. Nevertheless, several other authors have also suggested that alternative mechanisms, including DNA damage, hormonal disruption, oxidative stress, epigenetic modifications, and changes in cell adhesion and migration, can also lead to the development of malformations in zebrafish embryos [57, 58, 59]. Consequently, establishing and formalizing a protocol to investigate the potential adverse effects of pollutants on fish has been regarded as a foremost priority in our times. The Fish Embryo Acute Toxicity (FET) test, as outlined in the Organization for Economic Co-operation and Development (OECD) TG 236, stands out for its standardized and efficient approach to assessing the acute toxicity of chemicals in early-stage fish embryos [60]. This test plays a pivotal role in efficiently identifying potential hazards while adhering to ethical considerations by reducing the need for adult fish testing. Within the framework of this test, zebrafish offer a unique opportunity to capture several teratogenic toxicity indicators, encompassing crucial embryonic development events, such as cell movement during the gastrula stage, formation of brain regions, heartbeat, and blood circulation [61]. These indicators effectively serve as pertinent endpoints for toxicological evaluation.
2.2.2 Behavioral changes
The incidence of neuroactive substances in aquatic environments is increasing, giving rise to apprehensions regarding potential hazards to hydrobionts [62]. According to reports, approximately 70,000 identified neurotoxic chemicals exist, but only a tiny proportion, roughly 10%, have thoroughly recognized toxicological mechanisms [63]. Therefore, precise behavioral assays become imperative to comprehend neuronal signaling, identify emerging neuroactive compounds, and forecast their modes of operation. Neurotoxicity testing utilizes various model organisms, encompassing invertebrates such as fruit flies and nematodes and vertebrates such as chickens, mice, and other mammals. Nevertheless, invertebrates lack intricate neural structures, including myelin sheaths, which imposes limitations on in vivo neural activity research [64]. Furthermore, the substantial expenses and spatial demands associated with testing on mice and chickens considerably hinder the efficient screening of neurotoxic compounds in large quantities. Therefore, carefully selecting suitable model organisms is particularly important in neurotoxicity studies. In the realm of neurotoxicity research, the zebrafish has emerged as a highly potent model for investigating alterations in the nervous system from diverse perspectives [65]. It provides a comprehensive examination of chemical mechanisms of action. This efficacy is primarily attributed to its analogous central nervous system (CNS) arrangement, neurogenesis process, and specific responsiveness to pollutants, which closely mirror those observed in other vertebrates [66]. Moreover, the zebrafish possesses a notable advantage in its capacity to engage in unrestricted swimming approximately 5 days postfertilization, during which its juvenile brain tissue undergoes differentiation into distinct segments, such as telencephalon, diencephalon, mesencephalon, hindbrain, and rhomboencephalon [67]. This particular characteristic greatly facilitates the assessment of various aspects, including locomotion, learning, memory behavior, and responsiveness to photoelectric stimulation.
In the last 10 years, there has been a notable emphasis on using sophisticated video-tracking tools and data-analyzing software to enable efficient and rapid screenings of adult and larval zebrafish locomotion. This emphasis stems from the necessity to explore toxicological impacts on behavior, encompassing the investigation of fundamental motor responses, sensorimotor reactions, and learning and memory processes [68]. Various diverse tests have been devised to fulfill these objectives, such as the novel tank diving test, the social interaction task, the shoaling test, the aggression test, and the predator avoidance test. The novel tank diving test is a behavioral experiment that utilizes the concept of thigmotaxis, a commonly observed behavior in aquatic species, including zebrafish [69]. Thigmotaxis refers to the instinctual tendency of fish to seek comfort and safety by staying close to the walls or bottom of an unfamiliar tank or environment [70]. In this experiment, thigmotaxis is employed as a measure of anxiety or stress levels in zebrafish. Zebrafish are highly social animals, and their complex social behaviors are investigated through the social interaction task. This task provides valuable insights into various aspects of zebrafish sociability, including their preference for social interaction, the establishment of dominance hierarchies, and territorial behavior [71]. Researchers can gain significant information about their social dynamics and behavior by observing their interactions in a controlled setting. Like many other social animals, zebrafish exhibit aggressive behaviors when defending their territories or striving for dominance within their social group [72]. The aggression test, thus, is employed to study the aggressive tendencies of zebrafish, particularly during territorial disputes or competition for resources. Despite their small size, zebrafish demonstrate sophisticated predator avoidance strategies, which are evaluated through the predator avoidance test. This experiment aims to assess their ability to detect and respond to cues from predators, such as visual or chemical signals [73]. Understanding the neural pathways and sensory mechanisms involved in evading predation is crucial for uncovering evolutionarily conserved survival traits in prey animals.
2.2.3 DNA damage
Per Guideline Numbers 487 and 489 issued by the OECD, genotoxic effects refer to a broad spectrum of damage inflicted upon DNA or chromosomes [74, 75]. This damage encompasses various types, including, but not limited to, DNA breaks, deletions, adducts, nucleotide modifications, linkages, rearrangements, gene mutations, chromosome aberrations, and aneuploidy [76]. Nonetheless, genotoxic effects have also been associated with other health conditions, such as hematology disorders, liver disease, endocrine disorders, and autoimmune diseases [77]. Thus, identifying pollutants capable of inducing genetic damage in somatic and/or germ cells is essential, and for this purpose, genotoxicity tests play a crucial role. Zebrafish have become a highly esteemed model system for conducting genotoxicity studies. Notably, they possess orthologous genes with eukaryotic organisms, indicating a shared genetic similarity with other organisms containing cell nuclei [78]. Moreover, zebrafish encompass nearly all the genes relevant to various DNA repair pathways, rendering them exceptionally well-suited for comprehending the mechanisms of DNA repair and damage response [79]. The micronucleus and comet assays are two of the most frequently employed tests to evaluate mutagenic and genotoxic effects induced by chemical agents in zebrafish. The micronucleus test in fish is a cytogenetic assay that examines abnormal little nuclei frequencies in nucleated erythrocytes, a characteristic unique to fish compared to mammals [80]. The primary objective of this test is to detect the potential cytogenetic damage induced by pollutants. Specifically, it aims to identify substances that lead to micronuclei forming acentric chromosome fragments or entire chromosomes [81]. Research data indicate that various substances, such as nanomaterials, metals, effluents, pesticides, pharmaceuticals, alkylating agents, steroids, hormones, minerals, and polycyclic aromatic hydrocarbons, exhibit mutagenic properties by inducing micronuclei formation in fish erythrocytes [82, 83, 84, 85]. The comet assay, also known as single cell gel electrophoresis (SCGE) protocol, represents a widely used and well-established technique employed for the evaluation of DNA damage and DNA repair mechanisms. The fundamental principle underlying the comet assay lies in the fact that when DNA undergoes damage induced by genotoxic agents such as radiation or chemicals, it experiences fragmentation [86]. Consequently, during electrophoresis, these damaged DNA fragments move away from the nucleus, giving rise to a distinct “tail” formation, whereas undamaged DNA remains closer to the nucleus, forming the “head” of the comet structure. DNA damage is quantified by measuring various parameters, including DNA tail rate, tail moment, total area, tail length, and tail intensity [87]. However, the comet assay also enables the analysis of DNA repair mechanisms. Researchers can investigate the efficiency of DNA repair processes in different cell types or under various conditions by observing the rate at which the comet tail diminishes after DNA damage [88]. Based on the findings presented by Canedo and Rocha in 2021 [78], the comet assay has emerged as the most widely utilized method for identifying genetic material damage in zebrafish. This technique was more prevalent than other approaches, such as the 8-OHdG ELISA kit, RAPD-PCR, and qRAPDPCR [78]. Thus, despite the continuous development of novel techniques, data indicate that the comet assay remains the primary choice for assessing genetic material damage in zebrafish studies.
2.2.4 Histopathological damage
Histopathological studies are crucial in environmental toxicology, assessing pollutants’ impact on living organisms. These investigations involve a thorough microscopic examination of tissues, enabling researchers to identify and quantify cellular alterations and damage caused by toxic exposure. One significant advantage of histopathology is its capability to detect subtle tissue changes at early stages of toxicity, even before visible signs or clinical symptoms become apparent [89]. Moreover, by analyzing cellular and tissue changes, histopathology offers valuable insights into the health of populations in the wild and assesses the effectiveness of pollution control measures [90]. Zebrafish have garnered significant interest as a valuable model organism for conducting toxicological assays, encompassing the evaluation of administered compounds and environmental contaminants. This heightened attention stems from the remarkable physiological responses and histological features of zebrafish, which closely resemble those observed in mammals [91]. Furthermore, a significant advantage of utilizing zebrafish in histopathology is the ability to observe multiple organs on a single slide, facilitated by their small size [92]. Two distinct methodologies are employed to quantify tissue modifications in the organs of zebrafish. These methodologies involve the estimation of the histological alteration index and the calculation of tissue alteration prevalence. The first approach, known as the histological alteration index, assesses the average tissue alterations by employing a predefined list of potential changes, further categorized into three distinct levels of severity [93]. The second approach involves calculating the occurrence of tissue alterations in zebrafish organs. In other words, this method analyzes more tissue samples to determine the frequency of specific changes or abnormalities [85]. In general, these quantitative methodologies significantly contribute to enhancing comprehension of organ pathology. Nonetheless, their efficacy is contingent upon being accompanied by molecular techniques, such as immunohistochemistry, TUNEL assay, in situ hybridization, among other relevant methodologies.
2.2.5 Hematotoxicity
The evaluation of hematologic effects in environmental toxicology assumes paramount importance because of its potential to elucidate the adverse impacts of environmental contaminants on the blood and blood-forming tissues in living organisms. The hematologic system, comprising various blood cells and components, is critical in maintaining essential physiological functions within the body [94]. Any disruption in this intricate system can lead to severe health implications, including the onset of anemia, impairment of immune function, and heightened susceptibility to infections [95]. Consequently, a comprehensive examination of hematologic impacts is imperative in enhancing our comprehension of the potential risks posed by environmental contaminants. Zebrafish have become an exceptional model organism for exploring pollutants’ hematologic toxic effects. One of the key reasons for its prominence is its developmental hematopoiesis, which remarkably mirrors the hematopoietic system’s development in mammals [96]. In addition, this process is regulated by molecular pathways that are conserved and shared with higher vertebrates, further enhancing its utility for research in this field [97]. Nevertheless, akin to any model system, zebrafish are not exempt from limitations. A prominent challenge lies in obtaining sufficient blood volume from these organisms. Fortunately, some authors have endeavored to overcome this obstacle by developing innovative methodologies for obtaining larger volumes of blood [98, 99].
2.2.6 New trends
Transgenic zebrafish have emerged as an advantageous model within ecotoxicology. These genetically modified organisms originate by introducing exogenous genes, which may arise from diverse sources, including other animals or humans [100]. The integration of the transgene into the host genome can be accomplished through various methodologies, wherein the most prevalent approaches encompass the utilization of transposons or nucleases, such as Zinc Finger Nucleases (ZFNs), Transcription Activator-like Effector Nucleases (TALENs), and RNA-guided endonucleases such as CRISPR/Cas9 (Clustered Regularly Interspersed Short Palindromic Repeats Associated Protein 9) [101]. The utilization of transgenic organisms in the realm of environmental monitoring and toxicological studies presents a multitude of advantages. This methodology facilitates the swift acquisition of real-time outcomes, primarily achieved through detecting color and fluorescence signals [101, 102]. Moreover, by employing these organisms, researchers can also delve into the functions of genes that play critical roles in detoxification processes and responses to environmental stressors [102]. Specifically, this approach allows for the controlled disruption or alteration of genes in model organisms, leading to the observation of phenotypic changes that provide crucial insights into the roles of these genes in mitigating the effects of environmental toxins [102]. As outlined in the research conducted by Brito et al. in 2022 [101], existing literature describes 97 distinct transgenic zebrafish lines. Among these variants, the Tg(fli1: EGFP) strain commands the highest degree of utilization (comprising 16.02% with n = 33), succeeded by Tg(kdrl: EGFP; 5.34% with n = 11), Tg(cyp19a1b: GFP; 3.88% with n = 8), as well as both Tg(myl7: GFP) and Tg(hsp70: GFP; each accounting for 3.4% with n = 7). These transgenic zebrafish strains have demonstrated notable efficacy in the exploration of diverse toxicities encompassing vasotoxicity, neurotoxicity, systemic toxicity, hepatotoxicity, endocrine disruption, cardiotoxicity, immunotoxicity, hematotoxicity, ototoxicity, and pancreotoxicity [103]. As an illustration, the exposition of Tg(kdrl: EGFP) embryos to malachite green (MG) and leucomalachite green (LMG) dyes spanning 14 hours generated the aberration of intersegmental vessels (ISVs) within the upper trunk region dorsal longitudinal anastomotic vessels (DLAVs) and posterior cardinal veins (PAVs). This exposure also elicited perturbations in the spatial differentiation of the dorsal aorta (DA) and posterior cardinal vein (PCV) [104]. In addition, MeHg affected dopaminergic neurons and mitochondrial activity of dopaminergic neurons in the caudal hypothalamus region in exposed Tg(slc6a3: EGFP) and Tg(slc6a3: MITO-mCherry-NPT) zebrafish larvae of 120 hpf [105]. Hence, the amended data substantiate the significance of the Tg(kdrl: EGFP), Tg(slc6a3: EGFP), and Tg(slc6a3: MITO-mCherry-NPT) strains in the scrutiny of diverse biomarkers, facilitating the elucidation of mechanisms underlying vasotoxicity and neurotoxicity induced by various chemical agents or pollutants.
3. Conclusions
Zebrafish exhibit a sophisticated sex-determination system influenced by their environment, making them a highly relevant model for investigating reproductive and embryonic toxicity from exposure to endocrine-disrupting chemicals (EDCs). In addition, their well-established behavioral assays enable efficient screening of neurotoxic compounds and examination of neuronal signaling and behavior. Zebrafish also possess orthologous genes and proficient DNA repair pathways, which gives them a significant advantage in genotoxicity testing compared with other organisms. Consequently, their heightened suitability for detecting and evaluating DNA damage induced by diverse pollutants is noteworthy. In conjunction with the above-established techniques, emerging trends in environmental toxicology, such as genome editing methods (TALENs, ZFNs, and CRISPR-Cas9), introduce exciting new possibilities. These cutting-edge techniques facilitate studying detoxification processes and organismal responses to environmental stressors. One particularly promising avenue is the use of genetically engineered zebrafish as living biosensors. This approach holds the potential for real-time detection and reporting of environmental pollutants, revolutionizing our ability to monitor and respond to ecological hazards effectively.
References
- 1.
Stefanakis AI, Becker JA. A review of emerging contaminants in water: Classification, sources, and potential risks. In: McKeown AE, Bugyi G, editors. Impact of Water Pollution on Human Health and Environmental Sustainability. Hershey, PA: IGI Global; 2016. pp. 55-80. DOI: 10.4018/978-1-4666-9559-7.ch003 - 2.
La Farre M, Pérez S, Kantiani L, Barceló D. Fate and toxicity of emerging pollutants, their metabolites and transformation products in the aquatic environment. TrAC Trends in Analytical Chemistry. 2008; 27 (11):991-1007 - 3.
Relyea R, Hoverman J. Assessing the ecology in ecotoxicology: A review and synthesis in freshwater systems. Ecology Letters. 2006; 9 (10):1157-1171 - 4.
Asif N, Malik M, Chaudhry FN. A review of on environmental pollution bioindicators. Pollution. 2018; 4 (1):111-118 - 5.
Adhish M, Manjubala I. Effectiveness of zebrafish models in understanding human diseases—A review of models. Heliyon. 2023; 9 (3):E14557. DOI: 10.1016/j.heliyon.2023.e14557 - 6.
Parichy DM, Elizondo MR, Mills MG, Gordon TN, Engeszer RE. Normal table of postembryonic zebrafish development: Staging by externally visible anatomy of the living fish. Developmental Dynamics. 2009; 238 (12):2975-3015. DOI: 10.1002/dvdy.22113 - 7.
Akhter A, Rahaman M, Suzuki RT, Murono Y, Tokumoto T. Next-generation and further transgenerational effects of bisphenol a on zebrafish reproductive tissues. Heliyon. 2018; 4 (9) - 8.
Betancur-R R, Wiley EO, Arratia G, Acero A, Bailly N, Miya M, et al. Phylogenetic classification of bony fishes. BMC Evolutionary Biology. 2017; 17 :1-40 - 9.
Khan FR, Alhewairini SS. Zebrafish (Danio rerio) as a model organism. Current Trends in Cancer Management. 2018; 27 :3-18 - 10.
Tang KL, Agnew MK, Hirt MV, Sado T, Schneider LM, Freyhof J, et al. Systematics of the subfamily danioninae (Teleostei: Cypriniformes: Cyprinidae). Molecular Phylogenetics and Evolution. 2010; 57 (1):189-214 - 11.
Richards JG. Bony Fishes — Zebrafish. 2011 - 12.
Sanders GE. Zebrafish in biomedical research: Head and body: Anatomy. In: Cartner SC, et al, editors. The Zebrafish in Biomedical Research. Seattle, WA: Academic Press; 2020. pp. 77-79. DOI: 10.1016/B978-0-12-812431-4.00006-3 - 13.
Stoskopf MK. Fish Medicine. Philadelphia. Philadelphia: WB Saunders Comp; 1993 - 14.
Perry SF, Ekker M, Farrell AP, Brauner CJ. Fish Physiology: Zebrafish. Vol. 29. London, UK: Academic Press; 2010 - 15.
Lars B, Beata FG. Biology and research applications. In: D’Angelo LP, de Girolamo, editors. Laboratory Fish in Biomedical Research. London, UK: Academic Press; 2022. pp. 3-21 - 16.
Amanze D, Iyengar A. The micropyle: A sperm guidance system in teleost fertilization. Development. 1990; 109 (2):495-500 - 17.
Kimmel CB, Ballard WW, Kimmel SR, Ullmann B, Schilling TF. Stages of embryonic development of the zebrafish. Developmental Dynamics. 1995; 203 (3):253-310 - 18.
Wilson C. Aspects of larval rearing. ILAR Journal. 2012; 53 (2):169-178 - 19.
Hisaoka KK, Firlit CF. Further studies on the embryonic development of the zebrafish, Brachydanio rerio (Hamilton-Buchanan). Journal of Morphology. 1960; 107 (2):205-225 - 20.
Singleman C, Holtzman NG. Growth and maturation in the zebrafish, Danio rerio: A staging tool for teaching and research. Zebrafish. 2014; 11 (4):396-406 - 21.
Budick SA, O’Malley DM. Locomotor repertoire of the larval zebrafish: Swimming, turning and prey capture. Journal of Experimental Biology. 2000; 203 (17):2565-2579 - 22.
Buske C, Gerlai R. Shoaling develops with age in zebrafish (Danio rerio). Progress in Neuro-Psychopharmacology and Biological Psychiatry. 2011; 35 (6):1409-1415 - 23.
Harper C, Lawrence C. The Laboratory Zebrafish. Boca Raton, FL: CRC Press; 2016 - 24.
Darrow KO, Harris WA. Characterization and development of courtship in zebrafish, Danio rerio. Zebrafish. 2004; 1 (1):40-45 - 25.
Liu S, Leach SD. Zebrafish models for cancer. Annual Review of Pathology: Mechanisms of Disease. 2011; 6 :71-93 - 26.
Howe K, Clark MD, Torroja CF, Torrance J, Berthelot C, Muffato M, et al. The zebrafish reference genome sequence and its relationship to the human genome. Nature. 2013; 496 (7446):498-503 - 27.
Saad M, Cavanaugh K, Verbueken E, Pype C, Casteleyn C, Van Ginneken C, et al. Xenobiotic metabolism in the zebrafish: A review of the spatiotemporal distribution, modulation and activity of cytochrome P450 families 1 to 3. The Journal of Toxicological Sciences. 2016; 41 (1):1-11 - 28.
Goldsmith P. Zebrafish as a pharmacological tool: The how, why and when. Current Opinion in Pharmacology. 2004; 4 (5):504-512 - 29.
White RM, Sessa A, Burke C, Bowman T, LeBlanc J, Ceol C, et al. Transparent adult zebrafish as a tool for in vivo transplantation analysis. Cell Stem Cell. 2008; 2 (2):183-189 - 30.
Zon LI. Zebrafish: A new model for human disease. Genome Research. 1999; 9 (2):99-100 - 31.
Gerlai R. High-throughput behavioral screens: The first step towards finding genes involved in vertebrate brain function using zebrafish. Molecules. 2010; 15 (4):2609-2622 - 32.
Meeker ND, Trede NS. Immunology and zebrafish: Spawning new models of human disease. Developmental & Comparative Immunology. 2008; 32 (7):745-757 - 33.
McGrath P, Li CQ. Zebrafish: A predictive model for assessing drug-induced toxicity. Drug Discovery Today. 2008; 13 (9-10):394-401 - 34.
Hoo JY, Kumari Y, Shaikh MF, Hue SM, Goh BH. Zebrafish: A versatile animal model for fertility research. BioMed Research International. 2016; 2016 :1-20. DOI: 10.1155/2016/9732780 - 35.
Vining B, Ming Z, Bagheri-Fam S, Harley V. Diverse regulation but conserved function: SOX9 in vertebrate sex determination. Genes. 2021; 12 (4):486 - 36.
Valdivieso A, Ribas L, Monleón-Getino A, Orbán L, Piferrer F. Exposure of zebrafish to elevated temperature induces sex ratio shifts and alterations in the testicular epigenome of unexposed offspring. Environmental Research. 2020; 186 :109601 - 37.
Park CB, Kim GE, On J, Pyo H, Park JW, Cho SH. Sex-specific effects of bisphenol S with tissue-specific responsiveness in adult zebrafish: The antiandrogenic and antiestrogenic effects. Ecotoxicology and Environmental Safety. 2022; 229 :113102 - 38.
Heinrichs-Caldas W, Ikert H, Almeida-Val VMF, Craig PM. Sex matters: Gamete-specific contribution of microRNA following parental exposure to hypoxia in zebrafish. Comparative Biochemistry and Physiology Part D: Genomics and Proteomics. 2023; 47 :101090 - 39.
Marlatt VL, Bayen S, Castaneda-Cortès D, Delbès G, Grigorova P, Langlois VS, et al. Impacts of endocrine disrupting chemicals on reproduction in wildlife and humans. Environmental Research. 2022; 208 :112584 - 40.
Metcalfe CD, Bayen S, Desrosiers M, Muñoz G, Sauvé S, Yargeau V. An introduction to the sources, fate, occurrence and effects of endocrine disrupting chemicals released into the environment. Environmental Research. 2022; 207 :112658 - 41.
Niemuth NJ, Jordan R, Crago J, Blanksma C, Johnson R, Klaper RD. Metformin exposure at environmentally relevant concentrations causes potential endocrine disruption in adult male fish. Environmental Toxicology and Chemistry. 2015; 34 (2):291-296 - 42.
Jantzen CE, Toor F, Annunziato KA, Cooper KR. Effects of chronic perfluorooctanoic acid (PFOA) at low concentration on morphometrics, gene expression, and fecundity in zebrafish (Danio rerio). Reproductive Toxicology. 2017; 69 :34-42 - 43.
Akemann C, Meyer DN, Gurdziel K, Baker TR. Developmental dioxin exposure alters the methylome of adult male zebrafish gonads. Frontiers in Genetics. 2019; 9 :719 - 44.
Xiang D, Zhong L, Shen S, Song Z, Zhu G, Wang M, et al. Chronic exposure to environmental levels of cis-bifenthrin: Enantioselectivity and reproductive effects on zebrafish (Danio rerio). Environmental Pollution. 2019; 251 :175-184 - 45.
Gu J, Li L, Yin X, Liang M, Zhu Y, Guo M, et al. Long-term exposure of zebrafish to bisphenol F: Adverse effects on parental reproduction and offspring neurodevelopment. Aquatic Toxicology. 2022; 248 :106190 - 46.
Huang Y, Wang XL, Zhang JW, Wu KS. Impact of endocrine-disrupting chemicals on reproductive function in zebrafish (Danio rerio). Reproduction in Domestic Animals. 2015; 50 (1):1-6 - 47.
Silveira CR, Varela Junior AS, Corcini CD, Soares SL, Anciuti AN, Kütter MT, et al. Effects of Bisphenol a on redox balance in red blood and sperm cells and spermatic quality in zebrafish Danio rerio. Ecotoxicology. 2019; 28 :913-922 - 48.
de Oliveira VS, Marins K, Mendes AKB, Zamoner A, Leite GAA, Silva FRMB. In vivo exposure to pyriproxyfen causes ovarian oxidative stress and inhibits follicle maturation in zebrafish. Journal of Applied Toxicology. 2023; 43 (6):799-807. DOI: 10.1002/jat.4425 - 49.
Ye M, Chen Y. Zebrafish as an emerging model to study gonad development. Computational and Structural Biotechnology Journal. 2020; 18 :2373-2380 - 50.
Zhang C, Li D, Ge T, Han J, Qi Y, Huang D. 2, 4-Dichlorophenol induces feminization of zebrafish (Danio rerio) via DNA methylation. Science of the Total Environment. 2020; 708 :135084 - 51.
Ma X, Xiong J, Li H, Brooks BW, You J. Long-term exposure to neonicotinoid insecticide acetamiprid at environmentally relevant concentrations impairs endocrine functions in zebrafish: Bioaccumulation, feminization, and transgenerational effects. Environmental Science & Technology. 2022; 56 (17):12494-12505 - 52.
van der Ven LT, van den Brandhof EJ, Vos JH, Wester PW. Effects of the estrogen agonist 17β-estradiol and antagonist tamoxifen in a partial life-cycle assay with zebrafish (Danio rerio). Environmental Toxicology and Chemistry: An International Journal. 2007; 26 (1):92-99 - 53.
Wei P, Zhao F, Zhang X, Liu W, Jiang G, Wang H, et al. Transgenerational thyroid endocrine disruption induced by bisphenol S affects the early development of zebrafish offspring. Environmental Pollution. 2018; 243 :800-808 - 54.
Elizalde-Velázquez GA, Gómez-Oliván LM, Islas-Flores H, Hernández-Navarro MD, García-Medina S, Galar-Martínez M. Oxidative stress as a potential mechanism by which guanylurea disrupts the embryogenesis of Danio rerio. Science of the Total Environment. 2021; 799 :149432 - 55.
Escobar-Huerfano F, Elizalde-Velázquez GA, Gómez-Oliván LM, Orozco-Hernández JM, Rosales-Pérez KE, Islas-Flores H, et al. Environmentally relevant concentrations of fluconazole alter the embryonic development, oxidative status, and gene expression of NRF1, NRF2, WNT3A, WNT8A, NRD1, and NRD2 of Danio rerio embryos. Water Emerging Contaminants & Nanoplastics. 2022; 1 (1):4. DOI: 10.20517/wecn.2021.03 - 56.
Elizalde-Velázquez GA, Gómez-Oliván LM, García-Medina S, Hernández-Díaz M, Islas-Flores H, Galar-Martínez M, et al. Polystyrene microplastics mitigate the embryotoxic damage of metformin and guanylurea in Danio rerio. Science of the Total Environment. 2022; 852 :158503 - 57.
Casas-Hinojosa I, Gómez-Oliván LM, Gutierrez-Noya VM, Gracía-Medina S, Rosales-Pérez KE, Orozco-Hernández JM, et al. Integrative approach to elucidate the embryological effects of caffeine in Cyprinus carpio: Bioconcentration and alteration of oxidative stress-related gene expression patterns. Science of the Total Environment. 2023; 894 :165016. DOI: 10.1016/j.scitotenv.2023.165016 - 58.
del Carmen Ramírez-Montero M, Gómez-Oliván LM, Gutiérrez-Noya VM, Orozco-Hernández JM, Islas-Flores H, Elizalde-Velázquez GA, et al. Acute exposure to 17-α-ethinylestradiol disrupt the embryonic development and oxidative status of Danio rerio. Comparative Biochemistry and Physiology Part C: Toxicology & Pharmacology. 2022; 251 :109199 - 59.
Orozco-Hernández JM, Gómez-Oliván LM, Elizalde-Velázquez GA, Heredia-García G, Cardoso-Vera JD, Dublán-García O, et al. Effects of oxidative stress induced by environmental relevant concentrations of fluoxetine on the embryonic development on Danio rerio. Science of the Total Environment. 2022; 807 :151048 - 60.
OECD. Test No. 236: Fish Embryo Acute Toxicity (FET) Test, OECD Guidelines for the Testing of Chemicals, Section 2. Paris: OECD Publishing; 2013. DOI: 10.1787/9789264203709-en - 61.
McCollum CW, Ducharme NA, Bondesson M, Gustafsson JA. Developmental toxicity screening in zebrafish. Birth Defects Research Part C: Embryo Today: Reviews. 2011; 93 (2):67-114 - 62.
Duarte IA, Fick J, Cabral HN, Fonseca VF. Bioconcentration of neuroactive pharmaceuticals in fish: Relation to lipophilicity, experimental design and toxicity in the aquatic environment. Science of the Total Environment. 2022; 812 :152543 - 63.
Iqubal A, Ahmed M, Ahmad S, Sahoo CR, Iqubal MK, Haque SE. Environmental neurotoxic pollutants. Environmental Science and Pollution Research. 2020; 27 :41175-41198 - 64.
Lin W, Huang Z, Zhang W, Ren Y. Investigating the neurotoxicity of environmental pollutants using zebrafish as a model organism: A review and recommendations for future work. Neurotoxicology. 2023; 94 :235-244. DOI: 10.1016/j.neuro.2022.12.009 - 65.
Fitzgerald JA, Könemann S, Krümpelmann L, Županič A, Vom Berg C. Approaches to test the neurotoxicity of environmental contaminants in the zebrafish model: From behavior to molecular mechanisms. Environmental Toxicology and Chemistry. 2021; 40 (4):989-1006 - 66.
Kalueff AV, Stewart AM, Gerlai R. Zebrafish as an emerging model for studying complex brain disorders. Trends in Pharmacological Sciences. 2014; 35 (2):63-75 - 67.
Audira G, Sampurna BP, Juniardi S, Liang ST, Lai YH, Hsiao CD. A versatile setup for measuring multiple behavior endpoints in zebrafish. Inventions. 2018; 3 (4):75 - 68.
d’Amora M, Giordani S. The utility of zebrafish as a model for screening developmental neurotoxicity. Frontiers in Neuroscience. 2018; 12 :976 - 69.
Blaser RE, Rosemberg DB. Measures of anxiety in zebrafish (Danio rerio): Dissociation of black/white preference and novel tank test. PLoS One. 2012; 7 (5):e36931 - 70.
Walz N, Mühlberger A, Pauli P. A human open-field test reveals thigmotaxis related to agoraphobic fear. Biological Psychiatry. 2016; 80 (5):390-397 - 71.
Fontana BD, Müller TE, Cleal M, de Abreu MS, Norton WH, Demin KA, et al. Using zebrafish (Danio rerio) models to understand the critical role of social interactions in mental health and wellbeing. Progress in Neurobiology. 2022; 208 :101993 - 72.
Suriyampola PS, Shelton DS, Shukla R, Roy T, Bhat A, Martins EP. Zebrafish social behavior in the wild. Zebrafish. 2016; 13 (1):1-8 - 73.
Ladu F, Bartolini T, Panitz SG, Chiarotti F, Butail S, Macrì S, et al. Live predators, robots, and computer-animated images elicit differential avoidance responses in zebrafish. Zebrafish. 2015; 12 (3):205-214 - 74.
OECD. Test No. 489. In: Vivo Mammalian Alkaline Comet Assay, OECD Guidelines for the Testing of Chemicals, Section 4. Paris: OECD Publishing; 2016. DOI: 10.1787/9789264264885-en - 75.
OECD. Test No. 487: In Vitro Mammalian Cell Micronucleus Test, OECD Guidelines for the Testing of Chemicals, Section 4. Paris: OECD Publishing; 2023. DOI: 10.1787/9789264264861-en - 76.
Savale SK. Genotoxicity of drugs: Introduction, prediction and evaluation. Asian Journal of Biomaterial Research. 2018; 4 (6):1-29 - 77.
Mohamed SAKS, Upreti S, Rajendra SV, Dang R. Genotoxicity: Mechanisms, testing guidelines and methods. Global Journal of Pharmacy & Pharmaceutical Sciences. 2017; 1 (5):133-138 - 78.
Canedo A, Rocha TL. Zebrafish (Danio rerio) using as model for genotoxicity and DNA repair assessments: Historical review, current status and trends. Science of the Total Environment. 2021; 762 :144084 - 79.
Pei DS, Strauss PR. Zebrafish as a model system to study DNA damage and repair. Mutation Research/Fundamental and Molecular Mechanisms of Mutagenesis. 2013; 743 :151-159 - 80.
Benvindo-Souza M, Oliveira EAS, Assis RA, Santos CGA, Borges RE, e Silva DDM, et al. Micronucleus test in tadpole erythrocytes: Trends in studies and new paths. Chemosphere. 2020; 240 :124910 - 81.
Canedo A, de Jesus LWO, Bailão EFLC, Rocha TL. Micronucleus test and nuclear abnormality assay in zebrafish (Danio rerio): Past, present, and future trends. Environmental Pollution. 2021; 290 :118019 - 82.
Gajski G, Gerić M, Žegura B, Novak M, Nunić J, Bajrektarević D, et al. Genotoxic potential of selected cytostatic drugs in human and zebrafish cells. Environmental Science and Pollution Research. 2016; 23 :14739-14750 - 83.
D’Costa AH, Shyama SK, Praveen Kumar MK, Fernandes TM. Induction of DNA damage in the peripheral blood of zebrafish (Danio rerio) by an agricultural organophosphate pesticide, monocrotophos. International Aquatic Research. 2018; 10 :243-251 - 84.
Esteban-Sánchez A, Johann S, Bilbao D, Prieto A, Hollert H, Seiler TB, et al. Multilevel responses of adult zebrafish to crude and chemically dispersed oil exposure. Environmental Sciences Europe. 2021; 33 (1):1-19 - 85.
Elizalde-Velázquez GA, Rosas-Ramírez JR, Raldua D, García-Medina S, Orozco-Hernández JM, Rosales-Pérez K, et al. Low concentrations of ciprofloxacin alone and in combination with paracetamol induce oxidative stress, upregulation of apoptotic-related genes, histological alterations in the liver, and genotoxicity in Danio rerio. Chemosphere. 2022; 294 :133667 - 86.
Collins A, Møller P, Gajski G, Vodenková S, Abdulwahed A, Anderson D, et al. Measuring DNA modifications with the comet assay: A compendium of protocols. Nature Protocols. 2023; 18 (3):929-989 - 87.
Gajski G, Gerić M, Semren TŽ, Lovaković BT, Oreščanin V, Pizent A. Application of the comet assay for the evaluation of DNA damage from frozen human whole blood samples: Implications for human biomonitoring. Toxicology Letters. 2020; 319 :58-65 - 88.
Vodicka P, Vodenkova S, Opattova A, Vodickova L. DNA damage and repair measured by comet assay in cancer patients. Mutation Research/Genetic Toxicology and Environmental Mutagenesis. 2019; 843 :95-110 - 89.
Kaufmann W, Jacobsen MC. Examination of organ toxicity. In: Reichl FX, Schwenk M, editors. Regulatory Toxicology. 2nd ed. AG, Switzerland: Springer Cham; 2021. pp. 117-127. DOI: 10.1007/978-3-030-57499-4_32 - 90.
Reddy PB, Rawat SS. Assessment of aquatic pollution using histopathology in fish as a protocol. International Research Journal of Environment Sciences. 2013; 2 (8):79-82 - 91.
Raldua D, Pina B. In vivo zebrafish assays for analyzing drug toxicity. Expert Opinion on Drug Metabolism & Toxicology. 2014; 10 (5):685-697 - 92.
Borges RS, Pereira ACM, de Souza GC, Carvalho JCT. Histopathology of zebrafish ( Danio rerio ) in nonclinical toxicological studies of new drugs. In: Bozkurt Y, editor. Zebrafish in Biomedical Research. London, UK: IntechOpen; 2019 - 93.
Matus GN, Pereira BV, Silva-Zacarin EC, Costa MJ, Alves C, dos Santos A, et al. Behavior and histopathology as biomarkers for evaluation of the effects of paracetamol and propranolol in the neotropical fish species Phalloceros harpagos. Environmental Science and Pollution Research. 2018; 25 :28601-28618 - 94.
Goodman CC, Fuller KS, O’Shea RK. The hematologic system. In: Pathology for the Physical Therapist Assistant. Elsevier Saunders; 2012. pp. 387-450 - 95.
Kim JH, Yu YB, Choi JH. Toxic effects on bioaccumulation, hematological parameters, oxidative stress, immune responses and neurotoxicity in fish exposed to microplastics: A review. Journal of Hazardous Materials. 2021; 413 :125423 - 96.
Ellett F, Lieschke GJ. Zebrafish as a model for vertebrate hematopoiesis. Current Opinion in Pharmacology. 2010; 10 (5):563-570 - 97.
Gore AV, Pillay LM, Venero Galanternik M, Weinstein BM. The zebrafish: A fintastic model for hematopoietic development and disease. Wiley Interdisciplinary Reviews: Developmental Biology. 2018; 7 (3):e312 - 98.
Babaei F, Ramalingam R, Tavendale A, Liang Y, Yan LSK, Ajuh P, et al. Novel blood collection method allows plasma proteome analysis from single zebrafish. Journal of Proteome Research. 2013; 12 (4):1580-1590 - 99.
Zang L, Shimada Y, Nishimura Y, Tanaka T, Nishimura N. Repeated blood collection for blood tests in adult zebrafish. JoVE (Journal of Visualized Experiments). 2015; 102 :e53272 - 100.
Gordon JW, Ruddle FH. Integration and stable germ line transmission of genes injected into mouse pronuclei. Science. 1981; 214 (4526):1244-1246 - 101.
Brito RS, Canedo A, Farias D, Rocha TL. Transgenic zebrafish (Danio rerio) as an emerging model system in ecotoxicology and toxicology: Historical review, recent advances, and trends. Science of the Total Environment. 2022; 848 :157665 - 102.
Horzmann KA, Freeman JL. Making waves: New developments in toxicology with the zebrafish. Toxicological Sciences. 2018; 163 (1):5-12 - 103.
Garcia GR, Noyes PD, Tanguay RL. Advancements in zebrafish applications for 21st century toxicology. Pharmacology & Therapeutics. 2016; 161 :11-21 - 104.
Jang GH, Park IS, Lee SH, Huh TL, Lee YM. Malachite green induces cardiovascular defects in developing zebrafish (Danio rerio) embryos by blocking VEGFR-2 signaling. Biochemical and Biophysical Research Communications. 2009; 382 (3):486-491 - 105.
Cheng R, Jia Y, Dai L, Liu C, Wang J, Li G, et al. Tris (1, 3-dichloro-2-propyl) phosphate disrupts axonal growth, cholinergic system and motor behavior in early life zebrafish. Aquatic Toxicology. 2017; 192 :7-15