Abstract
The modern world is confronted with the pressing challenges of reducing carbon dioxide (CO2) emissions as the leading promoter of climate change while also addressing the energy and food crisis. Microalgae, being photosynthetic organisms, possess the unique ability to utilize solar energy for the conversion of CO2 into organic matter. Their ability to remove CO2 from the atmosphere proves advantageous in mitigating climate change. Algae have demonstrated effectiveness in wastewater treatment (WWT) by removing pollutants. In agriculture, microalgae offer a green alternative to chemical fertilizers, benefiting crop growth. The ease and cost-effectiveness of sampling and culturing microalgae contribute to their popularity. This chapter examines the sustainable applications of microalgae, including WWT, bioproduct recovery, bioremediation, energy production, agriculture, and biodiversity conservation. The examination of the growing global microalgae market, particularly in relation to biotechnological applications, was also reviewed as an indicator of the immense potential offered by microalgae.
Keywords
- climate change
- biodiversity loss
- microalgae
- sustainability
- ecosystem services
1. Introduction
Climate change is characterized by persistent modifications in Earth’s climate patterns, primarily attributed to anthropogenic factors such as the combustion of fossil fuels, deforestation, and industrial activities. The consequential rise in global temperatures and atmospheric carbon dioxide (CO2) levels has been unequivocally linked to far-reaching alterations in hydrological cycles, including precipitation and evaporation dynamics. Furthermore, climate change has been observed to engender an escalation in the magnitude and frequency of extreme weather phenomena [1]. These disturbances hold profound implications for biodiversity, exerting a significant negative impact on ecosystems worldwide. Consequently, climate change has emerged as a significant driver of biodiversity loss, exerting substantial impacts on ecosystems at a global scale [2]. One of the primary consequences of climate change is the loss of habitats, predominantly attributed to alterations in temperature and precipitation patterns. The changing climate disrupts ecosystems, transforming previously suitable habitats into unsuitable environments for numerous species. This loss of habitat contributes significantly to the ongoing decline in biodiversity.
Biodiversity encompasses the diversity of genes, species, and ecosystems found within a given area or across the planet. It includes the variation in genetic makeup within species, which provides the basis for adaptation and evolutionary processes. Species diversity, on the other hand, refers to the number and abundance of different species in a specific area, reflecting the richness and composition of biological communities. The impact of climate change on biodiversity is of great scientific concern, given the critical role biodiversity plays in supporting ecosystem stability, resilience, and functionality. In a study by Thomas et al. [3], a concerning outlook is presented, suggesting that climate change has the potential to drive the extinction of over a million terrestrial species within the next 50 years. This stark projection highlights the urgent need to address climate change as a primary driver of biodiversity loss. Implementing effective mitigation measures and adaptation strategies becomes imperative to safeguard biodiversity and ensure the long-term viability of ecosystems.
Microalgae, as photosynthetic unicellular microorganisms, play a crucial role in enhancing the productivity, biodiversity, and functioning of aquatic ecosystems. Particularly, phytoplankton, a subgroup of microalgae adapted to living in suspension in water, account for a substantial portion of the global net primary production in marine and freshwater environments, contributing approximately 49% [4]. The taxonomic diversity of microalgae is extensive, encompassing species that exhibit significant evolutionary divergence. Despite their small size, ranging from 0.2 to 200 μm, microalgae have a remarkable capacity to contribute to climate change mitigation through their ability to fix CO2 [5]. This process of carbon fixation by microalgae holds great potential for sequestering CO2 from the atmosphere, offering possibilities for sustainable utilization such as bioenergy production and the development of value-added products [6]. The significance of microalgae extends beyond their CO2-removing potential in combating climate change, as they possess remarkable capabilities to address various environmental challenges. Their capacity for biomonitoring enables effective assessment of water pollution levels, facilitating remediation efforts through bioremediation processes. Microalgae in wastewater treatment has garnered significant attention as a promising strategy with several notable benefits. This approach is characterized by its low energy demand, making it an energy-efficient option for treating wastewater. Microalgae exhibit remarkable adaptability and can thrive under various environmental conditions, allowing for effective wastewater treatment. Additionally, microalgae offer the potential to recover and transform nutrients present in wastewater into highly valuable bioactive compounds. This integrated approach not only addresses wastewater management but also presents an opportunity for resource recovery and the production of valuable products. The utilization of microalgae in wastewater treatment holds great promise in advancing sustainable practices and contributing to the circular economy [7]. Furthermore, microalgae contribute to the production of eco-friendly energy sources, offering a renewable and sustainable alternative to fossil fuels. Industries in the food and pharmaceutical sectors capitalize on the diverse biochemical composition of microalgae, utilizing them to produce various value-added products. Moreover, microalgae play a crucial role in promoting sustainable agriculture through various mechanisms. They enhance the growth of beneficial bacteria in the soil, contributing to a healthier soil microbiome. Microalgae help improve soil organic carbon content, which enhances soil fertility and nutrient availability for plants. They also have the ability to fix atmospheric nitrogen, making it accessible to plants and reducing the need for synthetic nitrogen fertilizers. Furthermore, they produce growth hormones that stimulate plant growth and development. Overall, the utilization of microalgae in agriculture holds significant potential for enhancing productivity, sustainability, and resilience in food production systems. Microalgae possess multifaceted attributes that are significant in addressing climate crises, including biodiversity loss, while promoting sustainable practices across different sectors [8].
This chapter aims to highlight the importance of microalgae in addressing climate change and biodiversity loss issues by uncovering the vast potential in promoting environmental sustainability. Through this comprehensive review, their potential in sectors like bioremediation and bioenergy, wastewater treatment, green agriculture for sustainable Earth, and value-added products in mitigating the biodiversity crisis will be highlighted. The chapter also examines the increasing global microalgae market. Figure 1 illustrates various applications of algae for achieving environmental sustainability against climate change and biodiversity loss.
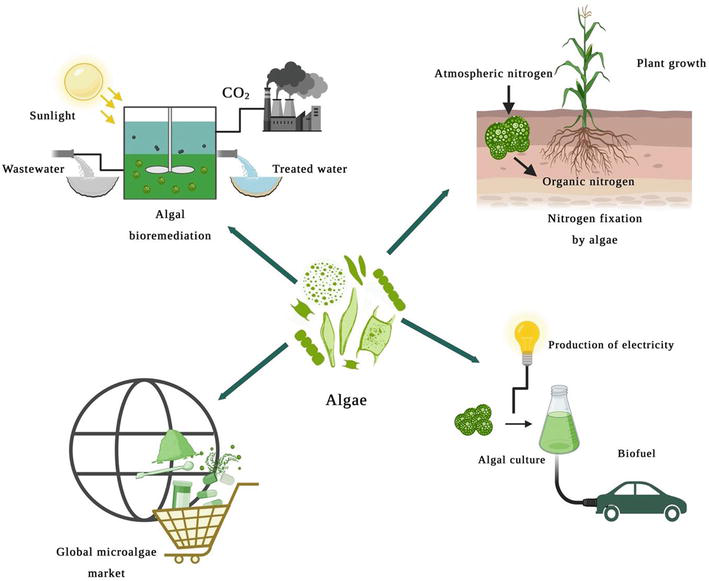
Figure 1.
Algal applications for sustainability.
2. Roles of microalgae-based systems for bioremediation and biofuel production
Energy production is currently confronted with a significant crisis due to a growing global population and the escalating demand for energy. The widespread use of fossil fuels has resulted in elevated levels of CO2 and other greenhouse gases in the atmosphere. Consequently, there is an urgent requirement for renewable and environmentally friendly fuel to satisfy the world’s energy needs. Algae can play a crucial role in meeting this demand by serving as a primary source of biofuel, owing to their advantageous qualities such as safety, non-competitiveness, and rapid growth [9]. Algal cells are known to contain a diverse array of valuable components, including fatty acids, carbohydrates, lipids, and proteins. Microalgae exhibit a remarkable capacity to accumulate lipids, with their oil content typically ranging from 20% to 50%. However, certain species have demonstrated an exceptional ability to accumulate lipids, reaching levels as high as 80%. These oil-rich algal species are considered highly promising to produce biofuels, as they offer a renewable alternative to conventional fossil fuels such as diesel, kerosene, and gasoline.
The utilization of algal biofuels brings forth several noteworthy benefits. Firstly, algal biofuels are environmentally advantageous due to their carbon neutrality. Their combustion releases an amount of CO2 equivalent to the CO2 they initially absorbed during growth, thus minimizing the net contribution of greenhouse gases to the atmosphere. This characteristic makes algal biofuels an attractive option for mitigating climate change and reducing overall carbon emissions. Moreover, algal biofuels offer considerable potential for economic sustainability. As nonfood feedstocks, microalgae cultivation for biofuel production avoids competition with food crops, thus alleviating concerns regarding food security. Additionally, the high oil content of certain algal species enables efficient extraction and conversion processes, resulting in improved energy output and cost-effectiveness [10]. Extensive research has been conducted on algal species known for their high oil content, particularly in relation to biofuel production. Among these species, several have gained recognition for their remarkable oil accumulation capabilities. One such species is
The production of biodiesel from microalgae involves a two-step process. The first step involves the extraction of lipids from microalgal cells, while the second step entails the transesterification of the extracted lipids using alcohol and a catalyst [13]. In addition to biodiesel production, microalgae have demonstrated their potential in the generation of other biofuels. Through photobiological processes, microalgae can be employed for the production of bio-hydrogen. Anaerobic digestion of microalgae can yield bio-methane, and fermentation processes can be utilized to produce bioethanol (Figure 2). These multifaceted applications of microalgae indicate their versatility as a sustainable resource for the production of different types of biofuels. With the strategic utilization of microalgae’s inherent capabilities, the development of environmentally friendly and renewable energy solutions becomes feasible [8].

Figure 2.
Biofuel production from microalgae.
Rahman et al. [14] conducted a study where a two-step transesterification process was employed to produce biodiesel from the microalgae species
Anaerobic digestion (AD) serves as a pivotal step in the generation of biogas and is a naturally occurring biological process with a long history. Organic components undergo biodegradation into biogas through the cooperative actions of diverse bacterial and archaeal species in oxygen-free conditions during AD [16]. Utilizing microalgae in AD has proven to be an efficient, practical, and cost-effective method for biogas production. While lignocellulosic wastes have traditionally been widely used in AD, their slow biodegradability can be attributed to the complex structure and nature of the biomass. Microalgae, in contrast, contain no lignin, leading to higher biodegradability compared to lignocellulosic biomass. Consequently, estimated methane yields from various common microalgae biomass are significantly higher than those from different wastes such as corn residues, wheat and rice straws, and sugarcane. In contrast to contemporary microalgae-based biodiesel production, which primarily utilizes high lipid-containing species, anaerobic digestion (AD) allows for the utilization of any microalgal species or residual biomass, regardless of their lipid content. This flexibility enables the inclusion of a wider range of microalgae in the AD process, either individually or through co-digestion. It has been observed that the generation of methane from microalgae through AD is more energy-intensive compared to microalgae-based biodiesel production. However, the advantage of AD lies in its ability to extract energy from microalgae biomass, regardless of its lipid content. Furthermore, the integration of biodiesel and biogas production from the same biomass can be achieved by utilizing lipid-extracted residues. These residues, obtained from biodiesel facilities, contain significant amounts of proteins and carbohydrates that can be biodegraded into biogas during the AD process. This integrated concept offers the potential for maximizing the energy yield and resource utilization from microalgae biomass [17].
Industrial and mining activities have led to the release of hazardous pollutants, with heavy metals being a significant concern that poses both environmental and health risks. The remediation of wastewater contaminated with heavy metals typically relies on conventional methods such as chemical precipitation and ion exchange [18]. However, these methods are often expensive and inefficient, particularly when it comes to meeting regulatory limits for heavy metal concentrations. In contrast, the utilization of microalgae for the treatment of heavy metal-loaded wastewater offers several advantages. Microalgae-based bioremediation exhibits potential applications across a wide range of environmental conditions, including varying pH levels, temperatures, the presence of other ions, and microalgal tolerance to different types of wastewater and heavy metals. This versatility makes microalgae a promising option for the bioremediation of heavy metal-contaminated wastewater. In addition to the capacity of being a natural feedstock particularly to produce biodiesel, microalgal biomass holds promise for the development of added-value bioproducts, including pigments, carbohydrates, and proteins [19].
Several studies have focused on the dual purpose of microalgal cultivation, aiming to simultaneously achieve wastewater treatment and biodiesel production. This approach capitalizes on the ability of microalgae to remove heavy metals from wastewater while also harnessing their lipid content for the production of biodiesel. By integrating these two objectives, researchers aim to create a sustainable and economically viable process that addresses both environmental concerns and the growing demand for renewable energy sources.
In a study conducted by Arora et al. [20], a synergistic approach combining bioremediation of arsenic and efficient biodiesel production was investigated. The selected microalgal strains, particularly
3. Roles of microalgae-based wastewater treatment and bioproducts recovery
Water is a fundamental and indispensable resource utilized as a primary raw material in a wide range of industries, encompassing pharmaceuticals, agriculture, health, beverage, agrochemicals, and oil and gas sectors, as well as for domestic usage. The release of contaminated water directly from these applications poses significant environmental risks and has become a growing concern due to the presence of diverse contaminants. Wastewater contains a variety of compounds that exist at concentrations that can be toxic to organisms. Additionally, important quantities of inorganic and organic nutrients are discharged into the surroundings, leading to elevated levels of chemical oxygen demand (COD) and biological oxygen demand (BOD). The excessive introduction of phosphorus (P) and nitrogen (N) into aquatic environments results in eutrophication, which engenders environmental issues like the generation of solid wastes and the emission of unpleasant substances into the atmosphere [21]. Furthermore, the proliferation of undesirable microorganisms facilitated by eutrophication poses a threat to aquatic organisms and compromises the potability of drinking water, thereby contributing to prevalent health concerns in areas adjacent to the discharge zone. Direct exposure to these toxic substances through inhalation, ingestion, or physical contact, even at minimal concentrations, may lead to substantial risks to human health and heighten the probability of cancer development [22].
In situations where water becomes contaminated and purification becomes imperative, it is crucial to select an appropriate treatment strategy to reach the purification aims. Conventional approaches commonly employed include various physical and chemical applications. Among these, chemical treatment stands out as one of the most effective wastewater treatment (WWT) approaches, as it aims to modify water characteristics by reducing turbidity, adjusting pH, and removing dissolved substances, thereby enhancing water quality. Although prominent techniques in chemical treatment such as chlorination, chloramination, UV light, and ozonation exhibit higher efficacy when utilized in secondary WWT where the objective is to decompose organic matter through microbial processes, their significant drawbacks are associated with high costs, limitations in dewatering, and extensive maintenance requirements [23]. In contrast, biological treatments depend on the metabolic processes of microbial to enzymatically degrade and transform wastewater pollutants into biomass and relevant gases, including CO2, methane (CH4), nitrogen (N2), and sulfur dioxide (SO2). This enzymatic degradation leads to a reduction in the levels of BOD in addition to a decrease in COD levels within the wastewater effluents, ultimately resulting in an enhancement of their quality. Biological wastewater treatment processes encompass the utilization of diverse microorganisms, such as bacteria, fungi, yeast, and microalgae, to facilitate the process of biodegradation. The primary objective of biological treatments is to establish a system effectively that is able to facilitate the treatment of discharged wastewater based on its inherent potential for decomposition [24].
The utilization of microalgae in biological WWT has emerged as a highly promising technology for the advanced treatment and recovery of nutrients from wastewater. This approach has garnered increasing attention in recent years since it presents a reliable solution for addressing liquid or solid waste generated by traditional methods while simultaneously transforming these wastes into valuable products with added benefits. Microalgae, which are oxygen-evolving photosynthetic microorganisms similar to plants, are widely present in various water environments, including fresh and marine water, as well as different types of wastewater. Extensive research has demonstrated the capacity of microalgae to effectively harness nutrients and thrive in wastewaters. This ability stems from their substantial requirement for nitrogen and phosphorous, alongside solar energy and either CO2 or organic substances as carbon sources. These essential elements are crucial for microalgae to synthesize vital components such as proteins, nucleic acids, and phospholipids while concurrently decreasing the concentration of these substances within the water [25].
The original purpose of employing microalgae in WWT was to address excessive nutrient levels in secondary effluent and mitigate the risk of eutrophication in natural water bodies. However, it is worth noting that the biomass production of microalgae during wastewater treatment holds considerable additional value. The harvested algal biomass can be utilized as a valuable feedstock in biorefinery processes or other applications, offering potential economic and environmental benefits [26]. Also, the symbiotic relationship between microalgae and bacteria is characterized by the utilization of resources and the exchange of byproducts. Microalgae engage in photosynthesis, utilizing CO2 to produce O2. Heterotrophic bacteria benefit from the generated oxygen, which they utilize to assimilate and degrade organic carbon, nitrogen, and phosphorus compounds. In turn, the aerobic metabolism of bacteria releases CO2, inorganic nitrogen, and phosphorus, which serve as nutrients for microalgae, facilitating their further photosynthetic activity. Microalgae-bacteria consortia not only effectively eliminate contaminants and nutrients but also generate valuable biomass that can be utilized in various applications. An illustrative instance involves the utilization of microalgae cultivated using cattle dairy wastewater, which has proven beneficial for fertilizing pasture. This application has resulted in elevated levels of essential minerals such as phosphorus, calcium, magnesium, and manganese, along with an increased content of dry matter [27].
Recent studies have revealed that the presence of algae can efficaciously help in the removal of nutrients from wastewater. For instance, Cai et al. [28] reported that the utilization of algae granules in synthetic wastewater exhibited a high level of efficiency in eliminating phosphorus, as well as recovering and reusing it from the resulting phosphorus-rich algae biomass. Moreover, aside from their effectiveness in capturing CO2 and removing nutrients from wastewater, microalgae have demonstrated potential as a viable source for energy generation. Furthermore, microalgae offer a promising approach for the removal and inactivation of pathogenic microorganisms due to the various mechanisms such as pH elevation, the production of microalgal toxins, and nutrient competition they employ. The process of photosynthesis, essential for microalgal growth, leads to an increase in pH and the enrichment of oxygen concentration in wastewater. As a result, the alterations in pH and dissolved oxygen levels can efficiently hinder the growth of pathogens in wastewater. In a study conducted by Mezzari et al. [29], it was found that the suppression of antibiotic-resistant
Microalgae have demonstrated significant potential in the production of various bioproducts, offering a diverse range of applications including biofuels, chemicals, food, feed, and pigments. The advantages of utilizing microalgae lie in their high productivity, the ability to cultivate them on marginal land using fresh or saltwater, which minimizes competition with food crops, and the potential to integrate biomass growth with the treatment of waste streams [31]. However, the extraction of these bioproducts can pose difficulties due to factors such as their solubility, small molecular weight, or low concentrations. In this regard, membrane filtration provides a flexible solution, enabling the separation of bioproducts from the growth media. Additionally, membrane filtration can assist in deliberate cell disruption, facilitating the release of intracellular products. Moreover, this technique can be utilized to harvest gases, such as oxygen, during the process. A study was conducted to explore the potential of thin stillage digestate as a growth medium for microalgae, with the objectives of investigating its effectiveness in removing nitrogen, phosphorus, and organic carbon and evaluating the potential of the resulting biomass for the production of biofuels and bioproducts. Thin stillage digestate was subjected to a pre-treatment process involving struvite recovery, which is a value-added product commonly used as a fertilizer. The treated digestate was then utilized as a growth medium for cultivating
Another valuable material produced by microalgae as a bioproduct is color pigments. The market demand for natural pigments derived from microalgae has significantly increased due to their remarkable coloring properties. These natural pigments are sought after as renewable and sustainable color enhancers for both food and feed applications. Additionally, they offer certain health benefits, making them highly desirable in the market. The increasing demand for natural pigments poses challenges due to the significant water and nutrient requirements in large-scale microalgae production, leading to high costs and hindering commercialization. To address these challenges, researchers have recently investigated the usage of wastewater for microalgae cultivation as a potential solution for natural pigment production. Furthermore, the potential application of anaerobic digestion at the end of the process to recover bioenergy from the residual biomass holds promise from both environmental and economic perspectives. In this regard, Jiang et al. [33] conducted a study to investigate the capacity of a diatom species,
In the realm of the bioeconomy, the adoption of innovative processes plays a crucial role in the production of bioproducts while simultaneously reducing the consumption of virgin resources. Microalgae-based WWT emerges as a significant contribution to this practice. This approach has attracted attention due to its minimal energy requirements, the adaptable growth characteristics of microalgae in various environmental conditions, and the potential to transform wastewater nutrients into bioactive compounds of significant value. These factors highlight the promising potential of microalgae-based wastewater treatment as a sustainable and resource-efficient solution in the bioeconomy.
4. Roles of microalgae-derived biofertilizer in the sustainability of green agriculture
In recent years, the rapid growth of the global population, along with the impacts of climate change, has placed significant strain on natural sources and placed a considerable burden on environmental conservation and agricultural sustainability. One of the critical areas of concern is ensuring an adequate food supply for the expanding population. The huge demand for potential food has placed substantial pressure on soil resources, leading to soil degradation. This degradation entails the loss of biodiversity and ecosystem services, and it is further exacerbated by the impacts of climate change, including altered precipitation patterns, increased frequency of extreme weather events, and shifts in soil temperature regimes. Soil degradation encompasses a range of adverse changes, such as reduced fertility, diminished organic matter content, and impaired water-holding capacity [34]. To address this challenge, the harnessing of fertilizers in agriculture plays a crucial role in ensuring an adequate food supply for the increasing global population. Fertilizers help bridge the nutrient gap in the soil, replenishing the necessary elements that are depleted through intensive farming practices. By supplementing the soil with nutrients, fertilizers contribute to increased crop yields and improved agricultural productivity. This, in turn, helps meet the rising food demands and ensures food security for the growing population [35].
Chemical fertilizers, produced through industrial means and offered in diverse commercial variations, frequently include inorganic micronutrients. Their primary purpose is to enhance crop yields and improve soil fertility. Nevertheless, the continuous application of fertilizers that are in inorganic form has been associated with negative outcomes. These include a decline in soil organic matter, increased soil acidity, degradation of soil physical and structural properties, contamination of groundwater and surface water, as well as potential carcinogenic effects for humans. The prolonged utilization of inorganic fertilizers has detrimental effects on the ecosystem, poses challenges to food security, and restricts the potential for land reuse in agricultural production [35]. Furthermore, the utilization of chemical fertilizer contributes to the release of various GHGs, with CO2 being the primary contributor. These emissions pose significant global concerns, not only impacting human health but also disrupting ecosystems. Considering these concerns, organic farming has gained considerable momentum as a rapidly growing agricultural technique on a global scale. This approach places a strong emphasis on utilizing biofertilizers to improve soil fertility while simultaneously avoiding the utilization of agrochemicals, genetically modified organisms, and synthetic substances commonly found as additives in food. This shift toward bio-based fertilizers is motivated by multiple factors, including the increasing demand for organic food in markets. To meet these market demands and produce organic food, farmers are increasingly adopting and implementing the use of bio-based fertilizers. This approach not only enhances soil fertility but also aligns with the principles of sustainable agriculture and environmental conservation [36].
Biofertilizers are intricately formulated products that leverage the symbiotic relationship between microorganisms and plants, or incorporate natural compounds from bacteria, fungi, and other organisms. They play a pivotal role in augmenting both the chemical and biological properties of the soil, thereby stimulating robust plant development and restoring soil fertility [37]. Extensive research has consistently highlighted the minimal impact of biofertilizers on human health and their ability to circumvent environmental pollution concerns. Notably, among the diverse array of biofertilizers, formulations rooted in photosynthetic organisms, particularly prokaryotic cyanobacteria and microalgae, have garnered significant recognition. These innovative formulations showcase remarkable aptitude in bolstering the bioavailability of nutrients, enriching soil fertility, and amplifying crop yields. A notable advantage lies in the biomass of photosynthetic organisms, which boasts a rich composition of proteins, carbohydrates, and vitamins, rendering them ideal for the development of eco-friendly fertilizers [38].
Microalgae represent a unique and diverse group of microorganisms, comprising both cyanobacteria (prokaryotic organisms) and eukaryotic organisms. They offer a multitude of advantages for addressing the complex agricultural landscape. These microscopic organisms have the potential to improve soil fertility, enhance plant growth, and reduce reliance on chemical fertilizers and pesticides. Using microalgal biomass as biofertilizer plays a beneficial role in soil nutrient cycling, facilitating the availability of essential nutrients for plant uptake (Figure 3). Upon direct contact of microalgal biomass with the soil, specific factors such as moisture, pH, and light percentages activate viable microalgae cells and sustain their metabolic activity. This process facilitates the capture of atmospheric N2 and supplies vital macronutrients and micronutrients that are essential for plant development. Microalgae also produce bioactive compounds such as phytohormones that positively influence plant growth and protect against phytopathogens and pests. Another noteworthy benefit is their ability to capture CO2 through photosynthesis, making them valuable for carbon sequestration. Furthermore, certain species of microalgae produce exopolysaccharides (EPS) that improve soil structure, enhancing water retention and aeration [39].
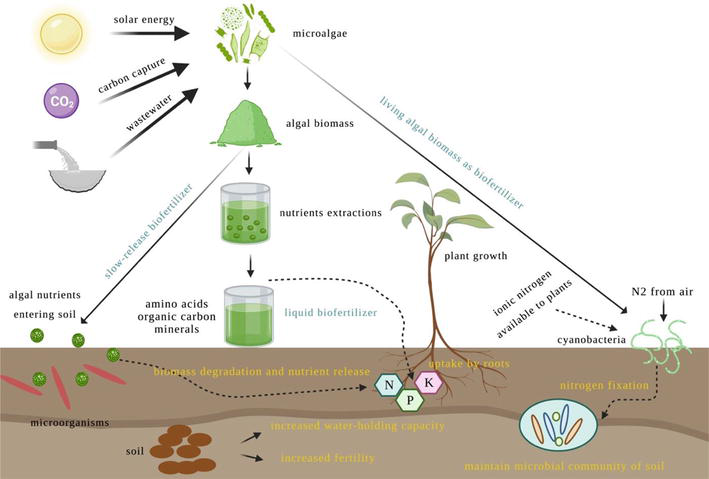
Figure 3.
Microalgae-derived biofertilizers on soil and crop improvement.
Additionally, microalgae can establish an endosymbiotic relationship with plant roots and other soil microorganisms. Extensive research on the symbiotic relationship between microalgae and plants was demonstrated in a study using a hydroponic system with
Moreover, measurements of photosynthetic pigments indicated no growth-related stress on the plant. Microalgae also form endosymbiotic relationships with bacteria, creating a mutually beneficial association that enables their survival and proliferation in various ecosystems. This symbiotic consortium presents opportunities for applications in WWT, reclamation of nutrient, production of feedstock, generation of biofuel, and biofertilizer development. Several studies have corroborated the efficacy of microalgae and other microorganisms, even in challenging environments such as deserts, in enhancing soil fertility. These beneficial microorganisms contribute to improved soil properties, including increased water retention; enhanced stability; pollutant removal; and the provision of a favorable substrate for plant growth [41].
A straightforward method for utilizing microalgae as a fertilizer involves directly applying the microalgal extract to the soil. However, to make this practice feasible and applicable, it is essential to optimize the energy requirements associated with it. Another alternative is the foliar spray method, where cellular extracts of microalgae are applied to the foliage of plants. This technique has also shown promising results, yielding profitable outcomes. Dineshkumar et al. [42] successfully implemented
Mahmoud et al. [44] conducted a study to investigate the impact of two different microalgal strains,
Microalgae offer a valuable source of organic carbon, addressing the issue of declining soil quality and soil fertility in croplands [46]. Via photosynthesis, microalgae generate extracellular polymeric substances (EPS), thereby enhancing the availability of organic carbon present in the soil. A pot experiment investigating the impact of microalgae biofertilizer reported a significant increase in soil organic carbon, which can be attributed to the photosynthetic activities of the microalgal species involved [47]. Furthermore, the assimilation of CO2 by microalgae can significantly increase soil organic carbon content. Research findings indicate that the utilization of biofertilizers results in a substantial rise of approximately 71.2% in soil organic carbon through the process of carbon sequestration [48]. Additionally, certain microalgae strains have the capacity to fix nitrogen, apart from their role in CO2 fixation. In cyanobacteria, for instance, while vegetative cells are responsible for CO2 fixation, specialized heterocystous cells perform nitrogen fixation, converting atmospheric nitrogen into ammonia [49].
One notable benefit of the application of microalgal biofertilizers is the enhancement of physical properties such as soil structure and water retention. Carillo et al. [50] observed that the utilization of microalgal biofertilizer led to augmented agglomeration of soil particles and increased water-holding capacity. Studies concerning cyanobacteria have highlighted the positive impact of biofertilizer application on both soil structure maintenance and fertility enhancement and subsequent improvements in crop yields [39].
Moreover, microalgal biofertilizers are able to contribute to the proliferation of bacterial community in soil. This, in turn, can lead to an overall enhancement of the microbial community in soil, thereby indirectly influencing plant growth. Marks et al. [51] demonstrated that the application of biofertilizers containing
5. Roles of microalgae-based product to prevent the global biodiversity crisis
Humanity is currently confronted with two pressing and closely interconnected environmental issues: climate change and biodiversity loss. As we step into a critical decade, the global efforts to address these challenges are becoming increasingly pivotal, with the development of ambitious strategies on both the international biodiversity and climate change fronts. Scientific evidence consistently highlights climate change as a major contributing factor to the current biodiversity loss and the primary factor of climate change is the emissions of greenhouse gases (GHG).
The emission of GHGs primarily stems from the combustion of fossil fuels, including coal, oil, and natural gas, in the automotive and industrial sectors. These activities contribute to carbon emissions during both the extraction and consumption processes. Also, the growing demand for meat and milk products has resulted in a substantial increase in livestock population and the conversion of extensive land areas into pastures and farmlands for livestock rearing. Ruminant animals, including cow, buffalo, and sheep, generate substantial quantities of methane through enteric fermentation during microbial digestion, thereby adding to the accumulation of GHGs in the atmosphere [52]. The production of 1 kg of meat requires 7 kg of grain and between 5000 and 20,000 L of water, whereas the production of 1 kg of wheat necessitates between 500 and 4000 L of water. Methane is also generated through the anaerobic fermentation of livestock manure. The importance of the need for transforming our animal farming industry by utilizing readily available plant-based ingredients, which can match the nutrient content of any sort of meat at a fraction of the cost, is seriously emphasized [53].
The main source of N2O emissions into the atmosphere (60%) originates from microbial activity on nitrogen-rich organic matter present in both uncultivated soils, besides wastewater. Human activities, particularly in agriculture, contribute to the remaining nitrous oxide emissions. The implementation of nitrogenous fertilizers on crops has a widespread practice aimed at increasing yields. However, it is common for most farmers to apply excessive amounts of these fertilizers. This practice results in N2O emissions from the soil, primarily through microbial processes such as nitrification and denitrification. Synthetic and organic fertilizers both contribute to increased N2 availability in the soil, which promotes microbial activity and subsequently leads to the release of N2O. However, organic fertilizer releases N2 into the soil at a slower pace compared to synthetic fertilizer. This gradual release enables plants to effectively absorb a larger proportion of the N2 as it becomes available. Synthetic fertilizer, on the other hand, rapidly releases N2 that cannot be immediately utilized by plants, thereby providing excess nitrogen to microbes for conversion into N2O. Currently, atmospheric CO2 concentration is higher than at any time in the past 2 million years, while methane and nitrous oxide levels are higher than in the last 800,000 years [54].
Shivanna [55] indicates that if climate change mitigation measures are not fully and precisely implemented, significant contractions in climatic ranges are likely to occur, resulting in a considerable decline in global biodiversity and ecosystem services by the end of the twenty-first century. In other words, taking immediate and stringent action to reduce GHG emissions holds the potential to mitigate the risk of widespread biodiversity loss among common and widely distributed species. Through the implementation of these measures, the concurrent decline in ecosystem services that are facilitated by these species can be effectively averted. Efforts to mitigate climate change focus on reducing GHG emissions through various means, such as transitioning to renewable energy sources, improving energy efficiency, implementing sustainable agricultural practices, and adopting cleaner technologies. Implementing nature-based solutions capitalizes on the intricate interplay between the climate system, oceans, land, and the encompassing natural environment. It is essential to manage this approach in a manner that preserves and sustains the multitude of contributions that nature provides to human societies and well-being, without jeopardizing their integrity [56]. Visions of nature-based strategies are emerging through the application of biotechnological transformations, encompassing the entire industrial chain. This worldwide trend embraces strategies that seek to diminish reliance on fossil fuel resources. In addition to designing safe foods, these strategies aim to significantly mitigate the impacts of the climate crisis on ecosystems and biodiversity loss. To achieve these goals, a substantial increase in the utilization of biological biomass is anticipated. Notably, the utilization of microalgal biomass to produce sustainable and environmentally friendly products stands out as one of the cleanest nature-based approaches that exhibit almost no GHG emissions [57]. Microalgal biomass has attracted significant interest in recent years due to its diverse range of potential applications. These applications include bioenergy production, wastewater treatment, industrial CO2 removal, and the production of biochemical compounds that offer benefits for human and animal health.
By harnessing microalgal biomass, it becomes possible to address multiple challenges such as mitigating climate change and reducing global biodiversity loss. One of the key advantages of microalgal biomass is its potential for bioenergy production. Microalgae can be cultivated to produce biofuels, which serve as a renewable and sustainable alternative to fossil fuels. This not only helps reduce GHG emissions but also decreases reliance on finite and environmentally damaging energy sources. WWT is another area where microalgal biomass shows promise. Microalgae have the ability to efficiently remove pollutants and nutrients from wastewater, thereby improving water quality and reducing the environmental impact of wastewater discharge. This contributes to the preservation of biodiversity in aquatic ecosystems. Additionally, microalgae have the capacity to capture and utilize CO2, making them a valuable tool for industrial carbon capture and utilization. By incorporating microalgae into industrial processes, CO2 emissions can be mitigated, helping to combat climate change and its detrimental effects on biodiversity. Furthermore, the production of biochemical compounds from microalgal biomass offers numerous health benefits for both humans and animals. These compounds can be used in pharmaceuticals, nutraceuticals, and other applications, promoting well-being and potentially reducing the reliance on synthetic alternatives that may have negative environmental impacts [58].
Another challenge is that climate change poses an escalating threat to soil degradation, which consequently results in the loss of biodiversity and crucial ecosystem services. Soils play a vital role in providing various ecosystem services, including sustainable plant production, water quality control, regulation of biological pests and diseases, nutrient and contaminant filtration, carbon storage, GHG regulation, waste detoxification, and recycling, as well as flood and climate change mitigation, among others. When soil degradation occurs, it impairs the capacity of the soil to support a diverse array of microorganisms, leading to a decline in soil biodiversity, complexity, and functionality. The loss of soil biodiversity and compromised ecosystem services can have implications for human health, as the soil plays a crucial role in supporting healthy plant growth, nutrient uptake, and the purification of water resources [59]. The utilization of microalgae for the bioremediation of degraded soils has been the subject of investigation, yielding promising outcomes. Contaminants, including heavy metals and pesticides, have a substantial impact on soil quality and the overall health of ecosystems. However, microalgae present an environmentally friendly and cost-effective solution for mitigating soil pollution. These microorganisms possess the ability to degrade pollutants and convert them into less harmful forms [60]. They enhance soil fertility by promoting overall microbial activity and facilitating beneficial microbial interactions. Microalgae support the growth of beneficial microbes in the soil, thereby influencing nutrient cycling and promoting nutrient availability for plants [61].
Moreover, numerous scientific studies have provided evidence for the probiotic-producing capabilities of various microalgae species. Microalgae have the ability to influence the microbial diversity in their environment through the secretion of various bioactive compounds including amino acids and extracellular polymeric substances (EPS), which function as a form of probiotic. For instance, Aydin et al. [30] investigated the application of
In summary, climate change is a pressing issue that affects all sectors of society and poses a threat to biodiversity, which represents the planet’s vitality. To preserve and restore biodiversity, it is crucial to limit deteriorative activities caused by mankind and address the global climate crisis. Mitigating climate change requires reducing GHG emissions, which are major drivers of climate change. The unsustainable use of fossil fuels for transportation, heating, and other industrial purposes, coupled with the increasing global population, has rapidly increased GHG emissions and triggered global warming and climate change. In addition, the harness of chemicals in agriculture and livestock farming to fulfill the rising food demand of the growing global population contributes significantly to greenhouse gas GHG emissions. To safeguard the present biodiversity and secure the future of life on our planet by mitigating the impacts of climate change, it is crucial to shift away from current energy sources toward renewable energy, enhance energy efficiency, adopt cleaner technologies, and establish sustainable agricultural practices. In this pursuit, prioritizing nature-based approaches is of utmost importance, as they are highly sustainable and result in minimal GHG emissions.
In recent years, biotechnological applications have emerged as a clean and environmentally friendly option by harnessing the beneficial microorganisms naturally present in the environment. Microalgae have shown great potential in combating climate change and preventing biodiversity loss through the production of renewable biofuels such as biodiesel and biogas. They have a renewable and GHG emission-free nature, making them an attractive option for energy production. Furthermore, utilizing these microorganisms in WWT, promoting sustainable agriculture through the usage of biofertilizers and biostimulants that enhance plant growth and soil biodiversity, and employing their bioproducts in various sectors such as health, cosmetics, and animal husbandry in the fight against climate change and biodiversity loss offer promising multifaceted applications. These approaches present adaptable and sustainable applications, offering a promising pathway to tackle climate change and mitigate global biodiversity loss.
6. Global market and economic analysis of microalgae technology for sustainability
Microalgae have garnered significant attention in the biomanufacturing field due to their extensive biodiversity and their capacity to synthesize a wide range of compounds. Beyond their role in carbon sequestration and oxygen production, microalgae offer a vast repertoire of metabolic pathways and biochemical reactions, allowing them to produce diverse and valuable compounds. The enormous biodiversity of microalgae encompasses numerous species with distinct genetic backgrounds, physiological characteristics, and biochemical capabilities. Microalgae can be engineered or selected for specific traits and optimized to produce various compounds. The ability of microalgae to perform complex biosynthetic pathways and their capacity for high-yield compound production make them valuable candidates for sustainable biomanufacturing. Their photosynthetic nature, coupled with the utilization of solar energy and CO2, further enhances their appeal as an environmentally friendly cell factory [62].
Microalgae exhibit a greater proportion of valuable cellular compositions in comparison to agriculturally cultivated biomass including edible food products and lignocellulosic biomass. Studies demonstrate the suitability of lipids produced from microalgae for conversion into alternative biofuels. Furthermore, microalgae play a significant role in the circular bioeconomy, as they contribute to transforming CO2 and water into oxygen through photosynthesis, thereby promoting the sustainability of the Earth [63]. In addition to their ecological benefits, microalgae biotechnology has emerged as a contributor by generating beneficial biomass for various applications. This refers to the utilization of microalgae to produce high-value compounds, such as proteins, lipids, polysaccharides, antioxidants, and pigments, which find applications in industries such as food, feed, cosmetics, pharmaceuticals, and specialty chemicals [64].
Numerous countries have recognized the significance of cultivating microalgae and have established a presence in the market for algae-based products over several years. Microalgae possess the unique capability to utilize solar energy, water, CO2, and inorganic nutrients to undergo photosynthesis, producing O2 while retaining biomass that is rich in proteins, vitamins, antioxidants, and other essential nutrients pivotal for human consumption. Among the commercially cultivated microalgal species worldwide,
The industry in Japan exhibits a strong emphasis on the biofuel application of microalgae and collaborates closely with the European Union (EU) in this regard. The Japanese market for microalgal biofuel is valued at approximately USD 1170 million, reflecting its significant size. Ongoing investments are being made in research and development programs, specifically targeting algal science, with a particular emphasis on biofuel production technologies. Furthermore, there is a notable drive to expand into various other microalgal markets, including foods, chemicals, and supplements. This diversification of applications indicates a broader utilization of microalgae beyond biofuel production [67]. In Europe, on the other hand, the industry is currently in the early stages of development compared to countries in the Eastern hemisphere. EU has recognized the utilization of microalgae to ensure the sustainability of the economy while effectively balancing different economically growth activities and concurrently preserving natural resources. This approach is driven by the aim to meet the demands of a rapidly growing population while ensuring the long-term viability of ecosystems and minimizing environmental impacts. The EU is actively exploring the potential of microalgae as a valuable resource for sustainable economic development [68].
EU is actively pursuing the development of a circular economy to address global issues including loss of biodiversity, climate crisis, environmental pollution, and waste management. This approach aims to establish systems that can combat these issues while protecting diversity. Unlike the linear economy model, which relies on resource exploitation and generates significant waste with minimal environmental considerations, the circular economy promotes sustainable practices that optimize resource utilization and minimize waste generation. The EU recognizes the importance of transitioning away from linear economy businesses and is dedicated to researching and implementing strategies that leverage microalgae’s potential. By this way, the EU is taking progressive steps toward achieving a more sustainable and environmentally conscious utilization of resources [69]. Recently, Europe has been at the forefront of the global
The present utilization of microalgae encompasses various applications, including food, feed, health-related products, and cosmetics. Microalgae biomass prices for these applications range from 5 to 500 €/kg, and the market size reaches up to 100 kt/year. Furthermore, microalgae demonstrate significant potential in sectors like biofuels, biofertilizers, wastewater treatment, and chemicals, where the biomass price is notably lower, below 5 €/kg. Despite the lower biomass price, these sectors offer immense market opportunities [64]. The most widely consumed microalgae species worldwide are
Microalgae are abundant sources of compounds with important applications in the health and cosmetic sectors. These organisms produce a variety of bioactive carbohydrates, antioxidants, pigments, and fatty acids, among other constituents. Carotenoids derived from microalgae play a significant role in health protection. Beta-carotene obtained from microalgal species
Among the diverse applications of microalgae, skin care has gained significant attention and utilization. Specifically, microalgal genera
Microalgae offer versatile applications in various fields, including the production of biomaterials. These biomaterials aim to decrease the dependence on conventional fossil-fuel-derived materials while offering enhanced properties for diverse applications. A notable area of interest is the development of bioplastics derived from microalgae, which offer environmental friendliness and biodegradability compared to current fossil-derived plastics. Microalgae possess the capability to produce a range of ingredients for the synthesis of bioplastics, such as polyester polymers named polyhydroxybutyrate (PHB). The improvement of PHB production efficiency by utilizing microalgal biomass derived from high-rate algal ponds holds the potential to decrease operational costs, thereby establishing microalgae as a sustainable and promising feedstock in the production of bioplastic in the foreseeable future. Nevertheless, it is crucial to acknowledge that microalgae-derived bioplastic production is currently in the comprehensive research phase and has not yet achieved commercialization. Further studies and advancements are necessary to optimize the production processes, scale up the technology, and address economic viability before widespread commercial implementation can be realized [75].
7. Conclusion
This chapter provides a comprehensive overview of the industrial applications of microalgae and their potential role in mitigating climate change and biodiversity loss. Although microalgae-derived approaches are still in the early stages of exploration, they hold significant promise as a future pathway for sustainable and green manufacturing. Microalgae exhibit the unique ability to harness solar energy and convert CO2 directly into organic carbon, enabling the production of a diverse array of high value bioproducts. Recent advancements in the field have positioned microalgae as promising sources for biofuels, biofertilizers, and the synthesis of complex macromolecules like proteins and nucleic acids that are valuable for human consumption and health. These bioactive molecules secreted by microalgae can have the ability to influence microbial diversity by enhancing the development of certain bacterial species. Furthermore, the advancement and widespread implementation of microalgae-based approaches present a viable means to mitigate the primary causal factor of climate change, GHG emissions. By effectively reducing GHG emissions at a global scale, the adverse impacts of the climate crisis on biodiversity loss and other ecological concerns can be alleviated. Furthermore, the growing global microalgae market signifies the promising potential of industrially developed and widely adopted microalgae-derived methods and bioproducts. These innovative solutions provide sustainable alternatives that can cater to a broader consumer base, contributing to the transition toward a more environmentally conscious and resilient future. However, the success and feasibility of microalgae-derived approaches rely on key factors such as efficient biomass accumulation and high yields of the target products. Continued research and development in this field are essential for unlocking the full potential of microalgae as a sustainable and environmentally friendly resource to mitigate global climate, food, and energy crisis.
References
- 1.
Lehmann P. Scientists’ warning on climate change and insects. Ecological Monographs. 2022 - 2.
Shin Y-J, Midgley GF, ERM A, Arneth A, DKA B, Chan L, et al. Actions to halt biodiversity loss generally benefit the climate. Global Change Biology. 2022; 28 (9):2846-2874. DOI: 10.1111/gcb.16109 - 3.
Thomas CD, Cameron A, Green RE, Bakkenes M, Beaumont LJ, Collingham YC, et al. Extinction risk from climate change. Nature. 2004; 427 (6970):145-148. DOI: 10.1038/nature02121 - 4.
Naselli-Flores L, Padisák J. Ecosystem services provided by marine and freshwater phytoplankton. Hydrobiologia. 2023; 850 (12-13):2691-2706 - 5.
Vu CHT, Lee HG, Chang YK, Oh HM. Axenic cultures for microalgal biotechnology: Establishment, assessment, maintenance, and applications. Biotechnology Advances. 2018; 36 (2):380-396. DOI: 10.1016/J.BIOTECHADV.2017.12.018 - 6.
Alami AH, Alasad S, Ali M, Alshamsi M. Investigating algae for CO2 capture and accumulation and simultaneous production of biomass for biodiesel production. Science of the Total Environment. 2021; 759 :143529. DOI: 10.1016/J.SCITOTENV.2020.143529 - 7.
Stabili L, Licciano M, Giangrande A, Caroppo C. Filtration of the microalga Amphidinium carterae by the polychaetes Sabella spallanzanii and Branchiomma luctuosum: A new tool for the control of harmful algal blooms? Microorganisms. 2022; 10 (1):156-169 - 8.
Roy A, Gogoi N, Yasmin F, Farooq M. The use of algae for environmental sustainability: Trends and future prospects. Environmental Science and Pollution Research. 2022; 29 (27):40373-40383. DOI: 10.1007/s11356-022-19636-7 - 9.
Schenk PM, Thomas-Hall SR, Stephens E, Marx UC, Mussgnug JH, Posten C, et al. Second Generation Biofuels: High-Efficiency Microalgae for Biodiesel Production. 2008; 1 :20-43. DOI: 10.1007/s12155-008-9008-8 - 10.
Slade R, Bauen A. Micro-algae cultivation for biofuels: Cost, energy balance, environmental impacts and future prospects. Biomass and Bioenergy. 2013; 53 :29-38. DOI: 10.1016/J.BIOMBIOE.2012.12.019 - 11.
Gouveia L, Ana, Oliveira C. Microalgae as a raw material for biofuels production. Journal of Industrial Microbiology & Biotechnology. 2009; 36 :269-274. DOI: 10.1007/s10295-008-0495-6 - 12.
Pradana YS, Sudibyo H, Suyono EA, Indarto, & Budiman, A. Oil algae extraction of selected microalgae species grown in monoculture and mixed cultures for biodiesel production. Energy Procedia. 2017; 105 :277-282. DOI: 10.1016/J.EGYPRO.2017.03.314 - 13.
Mondal M, Goswami S, Ghosh A, Oinam G, Tiwari ON, Das P, et al. Production of biodiesel from microalgae through biological carbon capture: A review. 3 Biotech. 2017; 7 . DOI: 10.1007/s13205-017-0727-4 - 14.
Rahman MA, Aziz MA, Al-khulaidi RA, Sakib N, Islam M. Biodiesel production from microalgae Spirulina maxima by two step process: Optimization of process variable. Journal of Radiation Research and Applied Sciences. 2017; 10 (2):140-147. DOI: 10.1016/J.JRRAS.2017.02.004 - 15.
Harun R, Danquah MK, Forde GM. Microalgal biomass as a fermentation feedstock for bioethanol production. Journal of Chemical Technology and Biotechnology. 2010; 85 (2):199-203. DOI: 10.1002/jctb.2287 - 16.
Harun R, Singh M, Forde GM, Danquah MK. Bioprocess engineering of microalgae to produce a variety of consumer products. Renewable and Sustainable Energy Reviews. 2010; 14 (3):1037-1047. DOI: 10.1016/J.RSER.2009.11.004 - 17.
Sialve B, Bernet N, Bernard O. Anaerobic digestion of microalgae as a necessary step to make microalgal biodiesel sustainable. Biotechnology Advances. 2009; 27 (4):409-416. DOI: 10.1016/J.BIOTECHADV.2009.03.001 - 18.
Joo SH, Tansel B. Novel technologies for reverse osmosis concentrate treatment: A review. Journal of Environmental Management. 2015; 150 :322-335. DOI: 10.1016/J.JENVMAN.2014.10.027 - 19.
Kafil M, Berninger F, Koutra E, Kornaros M. Utilization of the microalga Scenedesmus quadricauda for hexavalent chromium bioremediation and biodiesel production. Bioresource Technology. 2022; 346 :126665. DOI: 10.1016/J.BIORTECH.2021.126665 - 20.
Arora N, Gulati K, Patel A, Pruthi PA, Poluri KM, Pruthi V. A hybrid approach integrating arsenic detoxification with biodiesel production using oleaginous microalgae. Algal Research. 2017; 24 :29-39. DOI: 10.1016/J.ALGAL.2017.03.012 - 21.
Wang C, Luo D, Zhang X, Huang R, Cao Y, Liu G, et al. Biochar-based slow-release of fertilizers for sustainable agriculture: A mini review. Environmental Science and Ecotechnology. 2022; 10 :100167. DOI: 10.1016/J.ESE.2022.100167 - 22.
Abidli A, Huang Y, Ben Rejeb Z, Zaoui A, Park CB. Sustainable and efficient technologies for removal and recovery of toxic and valuable metals from wastewater: Recent progress, challenges, and future perspectives. Chemosphere. 2022; 292 :133102. DOI: 10.1016/J.CHEMOSPHERE.2021.133102 - 23.
Al-Tohamy R, Ali SS, Li F, Okasha KM, Mahmoud YAG, Elsamahy T, et al. A critical review on the treatment of dye-containing wastewater: Ecotoxicological and health concerns of textile dyes and possible remediation approaches for environmental safety. Ecotoxicology and Environmental Safety. 2022; 231 :113160. DOI: 10.1016/J.ECOENV.2021.113160 - 24.
Danso B, Ali SS, Xie R, Sun J. Valorisation of wheat straw and bioethanol production by a novel xylanase- and cellulase-producing Streptomyces strain isolated from the wood-feeding termite, Microcerotermes species. Fuel. 2022; 310 :122333. DOI: 10.1016/J.FUEL.2021.122333 - 25.
Pires JCM, Alvim-Ferraz MCM, Martins FG, Simões M. Wastewater treatment to enhance the economic viability of microalgae culture. Environmental Science and Pollution Research. 2013; 20 (8):5096-5105. DOI: 10.1007/s11356-013-1791-x - 26.
Zhou W, Li Y, Min M, Hu B, Zhang H, Ma X, et al. Growing wastewater-born microalga Auxenochlorella protothecoides UMN280 on concentrated municipal wastewater for simultaneous nutrient removal and energy feedstock production. Applied Energy. 2012; 98 :433-440. DOI: 10.1016/J.APENERGY.2012.04.005 - 27.
Lorentz JF, Calijuri ML, Assemany PP, Alves WS, Pereira OG. Microalgal biomass as a biofertilizer for pasture cultivation: Plant productivity and chemical composition. Journal of Cleaner Production. 2020; 276 :124130. DOI: 10.1016/J.JCLEPRO.2020.124130 - 28.
Cai W, Zhao Z, Li D, Lei Z, Zhang Z, Lee DJ. Algae granulation for nutrients uptake and algae harvesting during wastewater treatment. Chemosphere. 2019; 214 :55-59. DOI: 10.1016/J.CHEMOSPHERE.2018.09.107 - 29.
Mezzari MP, Prandini JM, Deon Kich J, Busi da Silva ML. Elimination of antibiotic multi-resistant salmonella typhimurium from swine wastewater by microalgae-induced antibacterial mechanisms. Journal of Bioremediation & Biodegradation. 2017; 08 (01). DOI: 10.4172/2155-6199.1000379 - 30.
Aydin S, Ünlü İD, Arabacı DN, Duru ÖA. Evaluating the effect of microalga Haematococcus pluvialis bioaugmentation on aerobic membrane bioreactor in terms of performance, membrane fouling and microbial community structure. Science of the Total Environment. 2022; 807 . DOI: 10.1016/j.scitotenv.2021.149908 - 31.
Ahmad A, Banat F, Alsafar H, Hasan SW. Algae biotechnology for industrial wastewater treatment, bioenergy production, and high-value bioproducts. Science of the Total Environment. 2022; 806 :150585. DOI: 10.1016/J.SCITOTENV.2021.150585 - 32.
Sayedin F, Kermanshahi-pour A, He QS, Tibbetts SM, Lalonde CGE, Brar SK. Microalgae cultivation in thin stillage anaerobic digestate for nutrient recovery and bioproduct production. Algal Research. 2020; 47 . DOI: 10.1016/j.algal.2020.101867 - 33.
Jiang J, Huang J, Zhang H, Zhang Z, Du Y, Cheng Z, et al. Potential integration of wastewater treatment and natural pigment production by Phaeodactylum tricornutum: Microalgal growth, nutrient removal, and fucoxanthin accumulation. Journal of Applied Phycology. 2022; 34 (3):1411-1422 - 34.
Gibbs HK, Salmon JM. Mapping the world’s degraded lands. Applied Geography. 2015; 57 :12-21. DOI: 10.1016/J.APGEOG.2014.11.024 - 35.
Nosheen S, Ajmal I, Song Y. Microbes as biofertilizers, a potential approach for sustainable crop production. Sustainability. 2021; 13 (4):1868-1888 - 36.
Chojnacka K, Moustakas K, Witek-Krowiak A. Bio-based fertilizers: A practical approach towards circular economy. Bioresource Technology. 2020; 295 :122223. DOI: 10.1016/J.BIORTECH.2019.122223 - 37.
Pirttilä AM, Mohammad Parast Tabas H, Baruah N, Koskimäki JJ. Biofertilizers and biocontrol agents for agriculture: How to identify and develop new potent microbial strains and traits. Microorganisms. 2021; 9 (4):817-834 - 38.
Abinandan S, Subashchandrabose SR, Venkateswarlu K, Megharaj M. Soil microalgae and cyanobacteria: The biotechnological potential in the maintenance of soil fertility and health. Critical Reviews in Biotechnology. 2019; 39 (8):981-998. DOI: 10.1080/07388551.2019.1654972 - 39.
Alvarez AL, Weyers SL, Goemann HM, Peyton BM, Gardner RD. Microalgae, soil and plants: A critical review of microalgae as renewable resources for agriculture. Algal Research. 2021; 54 :102200. DOI: 10.1016/J.ALGAL.2021.102200 - 40.
Özer Uyar GE, Mısmıl N. Symbiotic association of microalgae and plants in a deep water culture system. PeerJ. 2022; 10 :e14536. DOI: 10.7717/peerj.14536 - 41.
Aditya L, Mahlia TMI, Nguyen LN, Vu HP, Nghiem LD. Microalgae-bacteria consortium for wastewater treatment and biomass production. Science of the Total Environment. 2022; 838 :155871. DOI: 10.1016/J.SCITOTENV.2022.155871 - 42.
Dineshkumar R, Kumaravel R, Gopalsamy J, Mohammad N, Sikder A, Sampathkumar P. Microalgae as bio-fertilizers for rice growth and seed yield productivity. Waste and Biomass Valorization. 2018; 9 :793-800. DOI: 10.1007/s12649-017-9873-5 - 43.
Garcia-Gonzalez J, Sommerfeld M. Biofertilizer and biostimulant properties of the microalga Acutodesmus dimorphus . Journal of Applied Phycology. 2016;28 :1051-1061. DOI: 10.1007/s10811-015-0625-2 - 44.
Mahmoud SA, Abd El-Aty AM, Kandil H, Siam HS. Influence of different algal species application on growth of spinach plant ( Spinacia oleracea L.) and their role in phytoremediation of heavy metals from polluted soil. Plant Archives. 2019;19 :2275-2281 - 45.
Renuka N, Prasanna R, Sood A, Ahluwalia AS, Bansal R, Babu S, et al. Exploring the efficacy of wastewater-grown microalgal biomass as a biofertilizer for wheat. Environmental Science and Pollution Research. 2016; 23 :6608-6620. DOI: 10.1007/s11356-015-5884-6 - 46.
Renuka N, Guldhe A, Prasanna R, Singh P, Bux F. Microalgae as multi-functional options in modern agriculture: Current trends, prospects and challenges. Biotechnology Advances. 2018; 36 (4):1255-1273. DOI: 10.1016/J.BIOTECHADV.2018.04.004 - 47.
Yilmaz E, Sönmez M. The role of organic/bio–fertilizer amendment on aggregate stability and organic carbon content in different aggregate scales. Soil and Tillage Research. 2017; 168 :118-124. DOI: 10.1016/J.STILL.2017.01.003 - 48.
Zhu X, Gu C, Li X, Ye Z, Cao Z, Liu Y. Characterization of ITO-SiNx nanopores for single-biomolecular sensing. In: 2021 IEEE 16th International Conference on Nano/Micro Engineered and Molecular Systems (NEMS). IEEE; 2021. pp. 180-183 - 49.
Singh Chauhan D, Sahoo L, Mohanty K. Maximize microalgal carbon dioxide utilization and lipid productivity by using toxic flue gas compounds as nutrient source. Bioresource Technology. 2022; 348 :126784. DOI: 10.1016/J.BIORTECH.2022.126784 - 50.
Carillo P, Ciarmiello LF, Woodrow P, Corrado G, Chiaiese P, Rouphael Y. Enhancing sustainability by improving plant salt tolerance through macro-and micro-algal biostimulants. Biology. 2020; 9 (9):253-274. DOI: 10.3390/biology9090253 - 51.
Marks EAN, Montero O, Rad C. The biostimulating effects of viable microalgal cells applied to a calcareous soil: Increases in bacterial biomass, phosphorus scavenging, and precipitation of carbonates. Science of the Total Environment. 2019; 692 :784-790. DOI: 10.1016/J.SCITOTENV.2019.07.289 - 52.
Sejian V, Bhatta R, Malik PK, Madiajagan B, Al-Hosni YAS, Sullivan M, et al. Livestock as Sources of Greenhouse Gases and its Significance to Climate Change. In: Greenhouse Gases. London, UK: InTech; 2016. DOI: 10.5772/62135 - 53.
Pimentel D, Pimentel M. Sustainability of meat-based and plant-based diets and the environment. The American Journal of Clinical Nutrition. 2003; 78 (3):660S-663S. DOI: 10.1093/AJCN/78.3.660S - 54.
AR6 Climate Change. AR6 Climate Change: The Sixth Assessment Report on Climate Change. 2021 - 55.
Shivanna KR. Climate change and its impact on biodiversity and human welfare. Proceedings of the Indian National Science Academy. 2022; 88 :160-171. DOI: 10.1007/s43538-022-00073-6 - 56.
Girardin CAJ, Jenkins S, Seddon N, Allen M, Lewis SL, Wheeler CE, et al. Nature-based solutions can help cool the planet-if we act now. Nature. 2021; 593 (7858):191-194 - 57.
de Carvalho JC, Magalhães AI, de Melo Pereira GV, Medeiros ABP, Sydney EB, Rodrigues C, et al. Microalgal biomass pretreatment for integrated processing into biofuels, food, and feed. Bioresource Technology. 2020; 300 :122719. DOI: 10.1016/J.BIORTECH.2019.122719 - 58.
Mobin S, Alam F, Chowdhury. Environmental impact of algae-based biofuel production: A review. In: AIP Conference Proceedings. Vol. 2681, No. 1. 2022 - 59.
Smiraglia D, Ceccarelli T, Bajocco S, Salvati L, Perini L. Linking trajectories of land change, land degradation processes and ecosystem services. Environmental Research. 2016; 147 :590-600. DOI: 10.1016/J.ENVRES.2015.11.030 - 60.
Ossai IC, Ahmed A, Hassan A, Hamid FS. Remediation of soil and water contaminated with petroleum hydrocarbon: A review. Environmental Technology & Innovation. 2020; 17 :100526. DOI: 10.1016/J.ETI.2019.100526 - 61.
Leloup M, Nicolau R, Pallier V, Yéprémian C, Feuillade-Cathalifaud G. Organic matter produced by algae and cyanobacteria: Quantitative and qualitative characterization. Journal of Environmental Sciences. 2013; 25 (6):1089-1097. DOI: 10.1016/S1001-0742(12)60208-3 - 62.
Sun Z, Chen H, Sun L, Wang Q. Converting carbon dioxide to high value-added products: Microalgae-based green biomanufacturing. GCB Bioenergy. 2023; 15 (4):386-398 - 63.
Chew KW, Khoo KS, Foo HT, Chia SR, Walvekar R, Lim SS. Algae utilization and its role in the development of green cities. Chemosphere. 2021; 268 :129322. DOI: 10.1016/J.CHEMOSPHERE.2020.129322 - 64.
Fernández FGA, Reis A, Wijffels RH, Barbosa M, Verdelho V, Llamas B. The role of microalgae in the bioeconomy. New Biotechnology. 2021; 61 :99-107. DOI: 10.1016/J.NBT.2020.11.011 - 65.
Lim HR, Khoo KS, Chew KW, Chang CK, Munawaroh HSH, Kumar PS, et al. Perspective of Spirulina culture with wastewater into a sustainable circular bioeconomy. Environmental Pollution. 2021; 284 :117492. DOI: 10.1016/J.ENVPOL.2021.117492 - 66.
Li J, Liu Y, Cheng JJ, Mos M, Daroch M. Biological potential of microalgae in China for biorefinery-based production of biofuels and high value compounds. New Biotechnology. 2015; 32 (6):588-596. DOI: 10.1016/J.NBT.2015.02.001 - 67.
Murata A, Karwowski W. On the root causes of the Fukushima Daiichi disaster from the perspective of high complexity and tight coupling in large-scale systems. Symmetry. 2021; 13 (3):414 - 68.
Araujo P, Polesel DN, Hachul H, Rita L, Bittencourt A, Tufik S, et al. Oxygen saturation during sleep as a predictor of inflammation in anovulatory women. Sleep & Breathing. 2021, 2021; 25 (3):1247-1255. DOI: 10.1007/s11325-020-02233-8/Published - 69.
Barsanti L, Gualtieri P. Is exploitation of microalgae economically and energetically sustainable? Algal Research. 2018; 31 :107-115. DOI: 10.1016/J.ALGAL.2018.02.001 - 70.
Loke Show P. Global market and economic analysis of microalgae technology: Status and perspectives. Bioresource Technology. 2022; 357 . DOI: 10.1016/j.biortech.2022.127329 - 71.
Belay A. Biology and industrial production of Arthrospira (Spirulina). In: Handbook of Microalgal Culture: Applied Phycology and Biotechnology. 2013. pp. 339-358 - 72.
Guruvayoorappan C, Kuttan G. β-Carotene inhibits tumor-specific angiogenesis by altering the cytokine profile and inhibits the nuclear translocation of transcription factors in B16F-10 melanoma cells. Integrative Cancer Therapies. 2007; 6 (3):258-270. DOI: 10.1177/1534735407305978 - 73.
Sathasivam R, Ki JS. A review of the biological activities of microalgal carotenoids and their potential use in healthcare and cosmetic industries. Marine Drugs. 2018; 16 (1):26-57 - 74.
Morone J, Alfeus A, Vasconcelos V, Martins R. Revealing the potential of cyanobacteria in cosmetics and cosmeceuticals — A new bioactive approach. Algal Research. 2019; 41 :101541. DOI: 10.1016/J.ALGAL.2019.101541 - 75.
Abdo SM, Ali GH. Analysis of polyhydroxybutrate and bioplastic production from microalgae. Bulletin of the National Research Centre. 2019; 43 (1):97. DOI: 10.1186/s42269-019-0135-5