Abstract
In this chapter, we have studied a spatially homogeneous and anisotropic Kantowski-Sachs universe in the presence of Barrow Holographic Dark Energy in the background of Saez-Ballester scalar-tensor theory of gravitation. To find the exact solution of the SB field equations, we have assumed that the shear scalar is directly proportional to the expansion scalar. This assumption leads to relation between metric potentials of the models. We have discussed non-interacting and interacting cosmological models. Moreover, we have discussed several cosmological parameters such as energy densities of DM and DE (
Keywords
- Kantowski-Sachs
- Barrow holographic
- scalar-tensor theory
- dark energy model
- theory of gravitation
1. Introduction
The modern cosmological evidence [1, 2, 3, 4] indicated that there is an accelerated expansion. The responsible cause behind this accelerated expansion is a miscellaneous element having exotic negative pressure termed as Dark Energy (DE). The nature and the cosmological origin of DE are still enigmatic. To describe the phenomenon of DE, several models have been presented. According to several findings, DE should behave like a fluid with ‘negative pressure, counterbalancing the action of gravity, and speeding up the universe’ [5, 6]. The general methodology is to define the dynamics of the universe by assuming the source of DE is represented as a non-zero “cosmological constant
Hooft [16] has proposed a new dark energy model, known as the Holographic Dark Energy (HDE) model, which was based on the Holographic Principle (HP) and some features of quantum gravity theory. The holographic principle states that the number of degrees of freedom of a gravity-dominated system must vary along with the area of the surface bounding the system [17, 18]. For a system with size
2. Body of the manuscript
Barrow [29, 30] has recently found the possibility that the surface of a black hole could have a complex structure down to arbitrarily tiny due to quantum-gravitational effects. The above potential impacts of the quantum-gravitational space-time form on the horizon region would therefore prompt another black hole entropy relation, the basic concept of black hole thermodynamics. In particular
Here
where
Nandhida and Mathew [39] have considered the Barrow Holographic Dark Energy as a dynamical vacuum, with Granda-Oliveros (GO) length as IR cut-off and studied the evolution of cosmological parameters with the best-estimated model parameters extracted using the combined data-set of supernovae type Ia pantheon (SN-Ia) and observational Hubble’s data. Bhardwaj et al. [40] have studied statefinder hierarchy model for the BHDE. Adhikary et al. [41] have constructed a BHDE in the case of non-at universe in particular, considering closed and open spatial geometry and observed that the scenario can describe the thermal history of the universe, with the sequence of matter and DE epochs. Considering BHDE Sarkar and Chattopadhyay [42] reconstruct modified gravity as the form of background evolution and point out that the equation of state can have a transition from quintessence to phantom with the possibility of Little Rip singularity. Saridakis [43] has studied modified cosmology through spacetime thermodynamics and Barrow horizon entropy. Koussour et al. [44, 45] have investigated Bianchi type
Shamir and Bhatti [46] have analyzed anisotropic DE Bianchi type
3. Metric and SB field equations
We consider a homogeneous and anisotropic KK Universe described by the line-element
where
We assume that the Universe is filled by a DM without pressure with energy density
where
where
and energy conservation equations are
where
The SB field Eq. (5), for KK line-element Eq.(3) with the help of Eq.(4), can be written as
We can write the conservation Eq.(7) of the DM and BHDE as
where overhead dot (.) denotes ordinary differentiation with respect to cosmic time
The SB field eqs. (8)–(11) form a system of four (4) non-linear equations with seven (7) unknowns;
The average scale parameter of the KK Universe is given by
The spatial volume of the universe
The average Hubble parameter
The Deceleration Parameter (DP)
4. Solution of the field equations and cosmological models
Hence to find the exact solution of the field equations, we have to use some physically viable conditions; The shear scalar (
where
where
Now using eqs. (16), and (18), we get the exact solution
where
From eq. (2), the energy density of BHDE is
Thus, the metric corresponding to the metric potentials (20) can be written as
From eqs. (10), (11), (20), and (21), we found the skewness parameter (
The scalar field
where
The plot of DP (
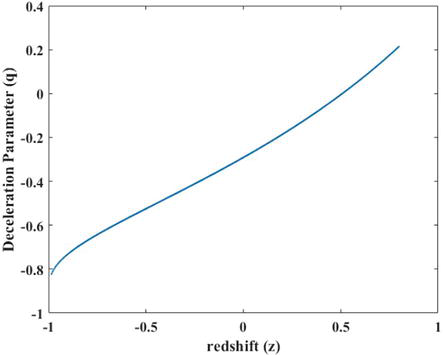
Figure 1.
Variation of deceleration parameter
4.1 Non-interacting BHDE in the SB cosmology
First, we consider that two fluids (DM and BHDE) do not interact with each other. Hence the conversation eq. (14), of the fluids may be conserved separately. The conservation eq. (14) of barotropic fluid leads to
whereas the conservation eq. (14) BHDE leads to
From eq. (21) by using eqs. (20), (21), and (23), we get the EoS parameter
where
The evolution of DM and BHDE densities with redshift (
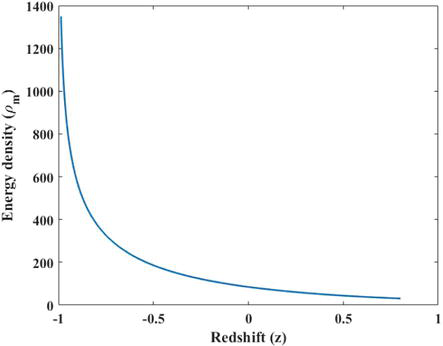
Figure 2.
Variation of energy density (
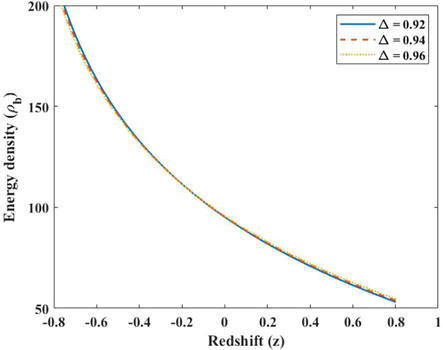
Figure 3.
Variation of energy density (
In Figure 4, we have plotted the behavior of skewness parameter (
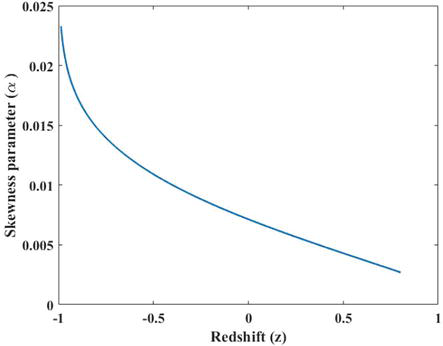
Figure 4.
Variation of skewness parameter (
In Figure 5, we observed the dynamics of the EoS parameter (
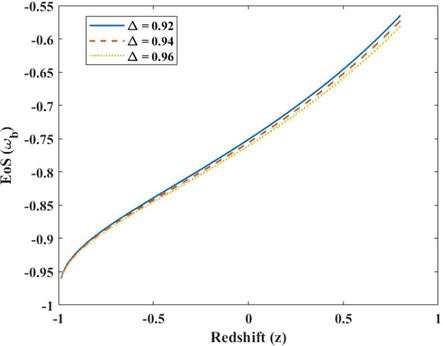
Figure 5.
Variation of equation of state parameter
4.2 ω b − ω b ' plane
Caldwell and Linder [56] have pointed out that the quintessence phase of DE can be separated into two distinct regions, that is, thawing (
where
Figure 6 shows the
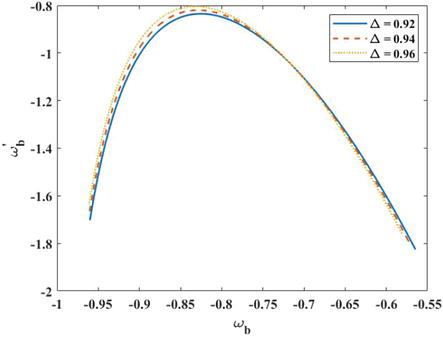
Figure 6.
Variation of
4.3 Stability analysis
We analyze now the stability of the obtained BHDE (non-interacting and interacting) models.
For our non-interacting BHDE model, squared speed sound
where
For the non-interacting model, Figure 7 shows the evolution of the SSS in terms of redshift (
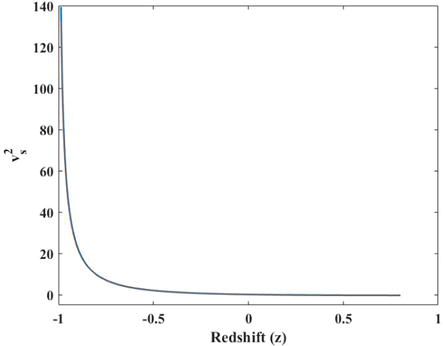
Figure 7.
Variation of squared sound speed
4.4 Interacting BHDE in the SB cosmology
In this case, we focus on the interaction between two dark fluids. Since the nature of both BHDE and DM is still unknown, there is no physical argument to exclude the possible interaction between them. Recently, some observational data shows that there is an interaction between dark sectors [57, 58]. Several authors [59, 60, 61] have investigated the signature of interaction between DE and DM by using optical,
whereas the conservation eq. (14) BHDE leads to
where
Now, from eqs. (20), (21), (23), (24), and (32), we found that the EoS parameter is
where
For interacting BHDE model, the EoS parameter (
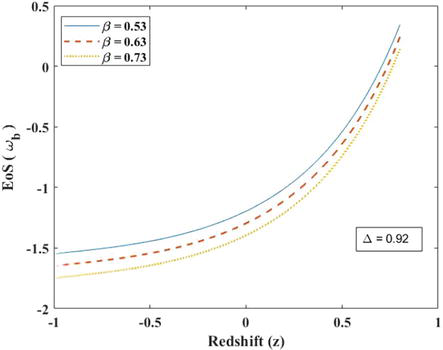
Figure 8.
Variation of
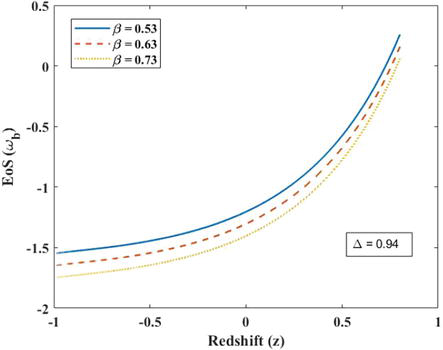
Figure 9.
Variation of
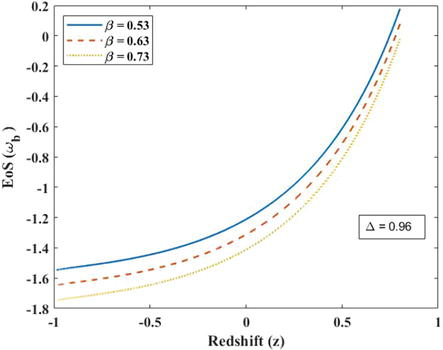
Figure 10.
Variation of
Figures 11–13 show the
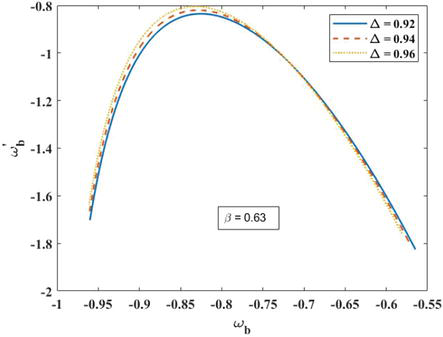
Figure 11.
Variation of
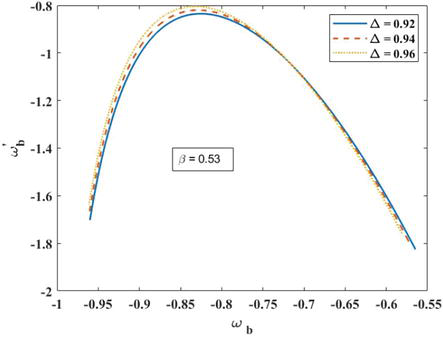
Figure 12.
Variation of
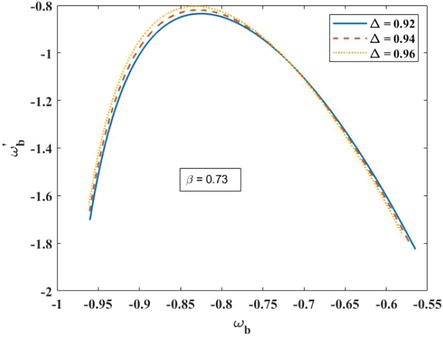
Figure 13.
Variation of
For our interacting BHDE model, Figure 14 shows the evolution of the SSS in terms of redshift (
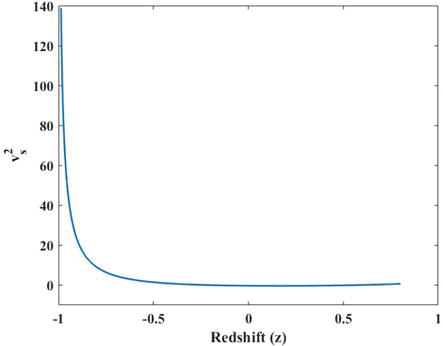
Figure 14.
Variation of squared sound speed (
4.5 Statefinder diagnostics
In this section, we focus on the diagnosis of the statefinder. The Hubble parameter
Figure 15 shows the evolutionary trajectories in
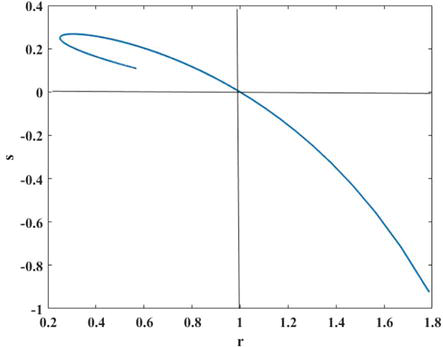
Figure 15.
Variation of statefinder parameters
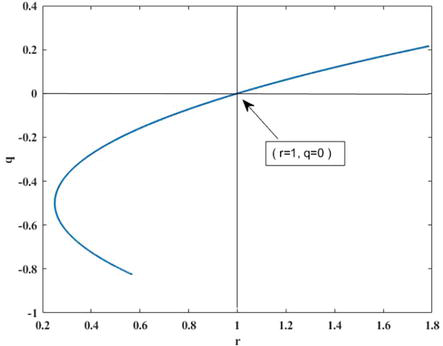
Figure 16.
Variation of statefinder parameters
4.6 Om-diagnostic
As a complementary to the statefinder parameters
where
The trajectory of Om diagnostics versus redshift (z) is shown in Figure 17. The trajectory reveals that the BHDE model shows initially a positive slope of the trajectory indicating that our model has phantom behavior and the negative slope of the trajectory indicates that our model behavior is quintessence in late time.
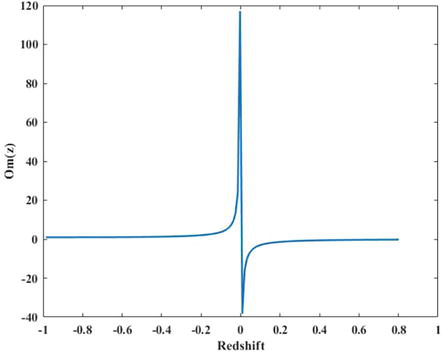
Figure 17.
Variation of statefinder parameters
5. Conclusions
In this chapter, we have investigated the accelerated expansion by assuming the BHDE Universe within the framework of SB scalar-tensor theory of gravity. We have investigated various cosmological parameters to analyze the viability of the models and our conclusions are the following:
The deceleration parameter (
For our non-interacting model, the energy densities of DM and BHDE are positive and increasing function of redshift
For interacting BHDE model, the EoS parameter starts from the matter dominated era, then it moves to the quintessence region (
It can be observed from Figures 5, 8–10 that the EoS parameter of our models in both non-interacting and interacting cases lie within the above observational limits which shows the consistency of our results with the above cosmological data. We have observed that our interacting BHDE model lies in the freezing region (
The behavior of
Finally, we can state that some of the preceding conclusions in KK BHDE model are good agreement with recent observations.
Conflict of interest
The authors declare that they have no known competing financial interests or personal relationships that could have appeared to influence the work reported in this chapter.
Data availability statement
This chapter has no associated data or the data will not be deposited.
References
- 1.
Riess AG et al. Observational Evidence from Supernovae for an Accelerating Universe and a Cosmological Constant. Astronomy Journal. 1998; 116 :1009 - 2.
Perlmutter S et al. Measurements of Ω and Λ from 42 High-Redshift Supernovae. The Astrophysical Journal. 1999; 517 :565 - 3.
Spergel DN et al. First-Year Wilkinson Microwave Anisotropy Probe (WMAP)* Observations: Determination of Cosmological Parameters. Astrophysical Journal of Supplement. 2003; 148 :175 - 4.
Tegmark M et al. Cosmological parameters from SDSS and WMAP. Physics Review D. 2004; 69 :103501 - 5.
Copeland EJ et al. Dynamics of dark energy. International Journal of Modern Physics D: Gravitation; Astrophysics and Cosmology. 2006; 15 :1753 - 6.
Ade PAR et al. Cosmological parameters. Astronomy and Astrophysics. 2016; 594 :A13 - 7.
Perlmutter S et al. Discovery of a supernova explosion at half the age of the Universe. Nature. 1998; 391 :51 - 8.
Schmidt BP et al. The high-z supernova search: measuring cosmic deceleration and global curvature of the universe using type Ia supernovae. The Astrophysical Journal. 1998; 507 :46 - 9.
Riess AG et al. Type ia supernova discoveries at z > 1 from the hubble space telescope: Evidence for past deceleration and constraints on dark energy evolution. The Astrophysical Journal. 2004; 607 :665 - 10.
Barreiro T et al. Quintessence arising from exponential potentials. Physics Review D. 2000; 61 :127301 - 11.
Caldwell et al. Phantom Energy: Dark Energy with ω < −1: Causes a Cosmic Doomsday. Physical Review Letters. 2003;91 :071301 - 12.
Bagla JS et al. Cosmology with tachyon field as dark energy. Physics Review D. 2003; 67 :063504 - 13.
Armendariz-Picon et al. Essentials of k-essence. Physics Review D. 2001; 63 :103510 - 14.
Cognola G et al. Dark energy in modified Gauss-Bonnet gravity: Late-time acceleration and the hierarchy problem. Physics Review D. 2006; 73 :084007 - 15.
Ferraro et al. Modified teleparallel gravity: Inflation without an inflaton. Physics Review D. 2007; 75 :084031 - 16.
‘t Hooft G. Dimensional Reduction in Quantum Gravity. 1993, arXiv e-prints, pp gr-qc/9310026 - 17.
Susskind L. The World as a Hologram. Journal of Mathematical Physics. 1995; 36 :6377 - 18.
Cohen A et al. Effective Field Theory, Black Holes, and the Cosmological Constant. Physical Review Letters. 1999; 82 :4971 - 19.
Hsu SD. Holographic Bound in Quantum Field Energy Density and Cosmological Constant. Physics Letters B. 2004; 594 :13 - 20.
Li M. The Interacting Generalized Ricci Dark Energy Model in Non-Flat Universe. Physics Letters B. 2004; 603 :1 - 21.
Zhang X, Wu FQ. Constraints on holographic dark energy from type Ia supernova observations. Physics Review D. 2005; 72 :043524 - 22.
Feng C et al. Journal of Cosmology and Astroparticle Physics. 2007; 09 :005 - 23.
Li M et al. Holographic dark energy models: a comparison from the latest observational data:. Journal of Cosmology and Astroparticle Physics. 2009; 06 :036 - 24.
Luongo O. A Thermodynamic Approach to Holographic Dark Energy. Advances High Energy Physics. 2017; 1 : 1424503. DOI: 10.1155/2017/1424503 - 25.
Setare MR. Interacting Dark Fluids in LRS Bianchi Type-II Universe. Physics Letters B. 2007; 654 :1 - 26.
Saridakis EN. Physics Letters B. 2008; 661 :335 - 27.
Singh CP, Srivastava M. European Physical Journal C: Particles and Fields. 2018; 78 :190 - 28.
Sadri E, Khurshudyan M. On An Interacting New Holographic Dark Energy Model: Observational constraints. International Journal of Modern Physics D: Gravitation; Astrophysics and Cosmology. 2019; 28 :1950152 - 29.
Saridakis EN. Barrow holographic dark energy: Physics Review D. 2000; 102 123525 - 30.
Pradhan A et al. A new class of holographic dark energy models in LRS Bianchi Type-I. International Journal of Modern Physics A. 2021; 36 :4 - 31.
Barrow JD. Physics Letters B. 2020; 808 :135643 - 32.
Saridakis SN, Basilakas S. The generalized second law of thermodynamics with Barrow entropy. The European Physical Journal C: 2000; 81 :644 - 33.
Anagnostopoulos FK et al. 2020. DOI: 10.48550/arXiv.2005.10302 - 34.
Barrow JD et al. Big Bang Nucleosynthesis constraints on Barrow entropy. Physics Letters B. 2021: 136134 - 35.
Srivastava S, Sharma UK. Barrow holographic dark energy with Hubble horizon as IR cutoff. International Journal of Geometric Methods in Modern Physics. 2021; 18 :2150014. - 36.
Mamon AA et al. Dynamics of an interacting barrow holographic dark energy model and its thermodynamic implications. The European Physical Journal Plus. 2021; 136 134 - 37.
Abreu EMC, Neto JA. Barrow black hole corrected-entropy model and Tsallis nonextensivity. Physics Letters B. 2020; 810 :135805 - 38.
Abreu EMC, Neto JA. Barrow fractal entropy and the black hole quasinormal modes. Physics Letters B. 2020; 807 :135602 - 39.
Nandhida PK, Mathew TK. Barrow holographic dark energy model with GO cut-off – An alternative perspective. International Journal of Modern Physics D. 2022; 31 :2250107 - 40.
Bhardwaj VK et al. Statefinder hierarchy model for the Barrow holographic dark energy. New Astronomy. 2021; 88 :101623 - 41.
Dudas E, et al. Slow and safe gravitinos. Physics Review D. 2021; 104 :123519 - 42.
Sarkar A, Chattopadhyay S. The barrow holographic dark energy-based reconstruction off (R) gravity and cosmology with Nojiri–Odintsov cutoff. International Journal of Geometric Methods in Modern Physics. 2021; 18 :2150148 - 43.
Saridakis EN. Modified cosmology through spacetime thermodynamics and Barrow horizon entropy. Journal of Cosmology and Astroparticle Physics. 2020; 07 :031 - 44.
Koussour M et al. Bianchi type-I Barrow holographic dark energy model in symmetric teleparallel gravity. International Journal of Modern Physics A. 2022; 37 :2250184 - 45.
Koussour M, Bennai M. Stability analysis of anisotropic Bianchi type-I cosmological model in teleparallel gravity. Classical and Quantum Gravity. 2022; 39 :105001 - 46.
Shamir MF, Bhatti AA. Canadian Journal of Physics. 2012; 90 :2 - 47.
Aditya Y, Reddy DRK. Anisotropic new holographic dark energy model in Saez–Ballester theory of gravitation. Astrophysics and Space Science. 2018; 363 :207 - 48.
Jawad A et al. Cosmological consequences and thermodynamics of modified gravity with extended nonminimal derivative couplings. International Journal of Modern Physics D. 2019; 28 :1950146 - 49.
Vijaya Santhi M, Sobhan Babu Y. Bianchi type-III Tsallis holographic dark energy model in Saez–Ballester theory of gravitation. European Physical Journal C. 2020; 80 :1198 - 50.
Vijaya Santhi M, Sobhan Babu Y. Tsallis holographic dark energy models in Bianchi type space time. New Astronomy. 2021; 89 :101648 - 51.
Sobhanbabu Y, Vijaya Santhi M. Kantowski–Sachs Tsallis holographic dark energy model with sign-changeable interaction. The European Physical Journal C. 2021; 81 :1040 - 52.
Sharif M, Majid A. Isotropic and complexity-free deformed solutions in self-interacting Brans–Dicke gravity. International Journal of Modern Physics. 2022; 31 :2240003 - 53.
Pradhan A et al. FRW cosmological models with Barrow holographic dark energy in Brans–Dicke theory. International Journal of Geometric Methods in Modern Physics. 2022; 19 :2250106 - 54.
Collins B et al. Exact Spatially Homogeneous Cosmologies. General Relativity and Gravitation. 1980; 12 :805 - 55.
Tiwari RK et al. Cosmological models with viscous fluid and variable deceleration parameter. European Physical Journal Plus. 2017; 132 :1 - 56.
Caldwell RR, Linder EV. The Limits of Quintessence. Physical Review Letters. 2005; 95 :141301 - 57.
Bertolami O et al. Dark energy–dark matter interaction and putative violation of the equivalence principle from the Abell cluster A586. Physics Letters B. 2007; 654 :165 - 58.
Bertolami O et al. The Abell cluster A586 and the detection of violation of the equivalence principle. General Relativity and Gravitation. 2009; 41 :2839 - 59.
Abdalla E et al. Signature of the interaction between dark energy and dark matter in galaxy clusters. Physics Letters B. 2009; 673 :107 - 60.
Abdalla E et al. Astrophysical uncertainties of dark matter direct detection experiments. Physical Review D. 2010; 82 :023508 - 61.
Sobhanbabu Y, Vijaya Santhi M. Anisotropic new agegraphic dark energy model with sign-changeable interaction in a scalar-tensor theory. The European Physical Jounal Plus. 2022; 137 :753 - 62.
Alam U et al. Exploring the Expanding Universe and Dark Energy using the Statefinder Diagnostic. Monthly Notices of the Royal Astronomical Society. 2003; 344 :1057 - 63.
Sahni V et al. Statefinder—A new geometrical diagnostic of dark energy. JETP Letters. 2003; 77 :201 - 64.
Aghanim N et al. Planck 2018 results. VI. Cosmological parameters. arXiv:1807.06209v2. 2018 - 65.
Zunckel C. Consistency Tests for the Cosmological Constant. Physical Review Letters. 2008; 101 :181301 - 66.
Shahalam M et al. Om diagnostic applied to scalar field models and slowing down of cosmic acceleration. Monthly Notices of the Royal Astronomical Society. 2015; 448 :2948 - 67.
Capozziello S. Model-independent constraints on dark energy evolution from low-redshift observations. Monthly Notices of the Royal Astronomical Society. 2019; 484 :4484