1. Introduction
The physics of gamma rays, which occupy the highest energy end of the electromagnetic spectrum, is intriguing and complex. Although gamma rays are most often linked with the most powerful and explosive phenomena in the cosmos, they also have significant consequences and uses on our home planet. This introductory chapter tries to demystify gamma rays, explaining their existence, origin, and the countless ways in which they impact and contribute to different scientific areas.
The gamma ray is the highest frequency and most powerful kind of electromagnetic energy. Gamma rays are electromagnetic radiation with a frequency of more than 10 exahertz and a wavelength shorter than 10 picometers, making them distinct from other forms of electromagnetic radiation [1].
Gamma rays’ origins are just as interesting as their characteristics. The decay of radioactive nuclei, also known as gamma decay, is a major contributor to this kind of radiation because it occurs when an unstable atomic nucleus releases a gamma ray as a means of dissipating its excess energy. This phenomenon is fundamental to comprehending many radioactive processes occurring on our planet [2, 3].
Also, gamma rays may come from sources other than Earth. These very powerful rays may be found in abundance throughout the universe. The ubiquitous gamma-ray background is mostly fueled by violent cosmic phenomena, including supernovae, the explosive death of big stars, and the interactions surrounding black holes and neutron star mergers. These astronomical occurrences reveal the tremendous power and rapid processes taking place in the depths of space [4].
The unique ability of gamma rays to interact with matter sets them different from other electromagnetic waves. Their enormous intensity enables them to penetrate materials more deeply than X-rays, making them a weapon of great usefulness and peril. This penetrating strength is used in radiation treatment for cancer, which destroys cancer cells [5]. However, the ionizing nature of gamma rays, which may disrupt chemical bonds and cause biological damage, presents considerable hurdles in terms of safety and radiation protection [5].
Scintillation detectors, semiconductor detectors, and Geiger-Müller tubes are only some of the specialized pieces of kit needed to detect and measure gamma rays. Due to the tremendous penetrating force and energy of gamma rays, these instruments are essential to their investigation.
Overall, gamma rays constitute a noteworthy and strong component of the electromagnetic spectrum [6]. Their unusual penetrating force and high energy, together with their generation from both terrestrial and cosmic processes, set them apart from other kinds of electromagnetic radiation, and have made them a topic of continuing interest and research in the domains of physics, astronomy, and medicine.
2. Applications and benefits
The ongoing investigation of gamma rays has been essential in uncovering novel and captivating prospects inside several scientific domains. An area that shows significant potential is the development of gamma ray lasers, which have the capability to produce gamma radiation beams with great coherence, similar to laser beams in the visible spectrum. These gadgets have the potential to revolutionize several fields such as medical imaging, security scanning, manufacturing, and other relevant applications. Simultaneously, enhanced comprehension of gamma ray interactions facilitates progress in radiation therapy, wherein procedures such as gamma knife surgery contribute to the treatment of previously incurable tumors by precisely targeting the affected tissue [7, 8]. The ongoing investigation of gamma rays has been essential in uncovering novel and captivating prospects inside several scientific domains. An area that shows significant potential is the development of gamma ray lasers, which have the capability to produce gamma radiation beams with great coherence, similar to laser beams in the visible spectrum. These gadgets have the potential to revolutionize several fields such as medical imaging, security scanning, manufacturing, and other relevant applications. Simultaneously, enhanced comprehension of gamma ray interactions facilitates progress in radiation therapy, wherein procedures such as gamma knife surgery contribute to the treatment of previously incurable tumors by precisely targeting the affected tissue.
Positron emission tomography (PET) scans are a prominent use of gamma rays within the field of diagnostic imaging (Figure 1). PET scans employ a radioactive tracer, often a type of glucose, which is injected into the patient’s bloodstream. The tracer releases positrons that engage in interactions with electrons inside the body, leading to the subsequent emission of gamma rays [9]. Subsequently, the PET scanner captures and detects these rays, generating intricate visual representations of the interior anatomical systems inside the body.
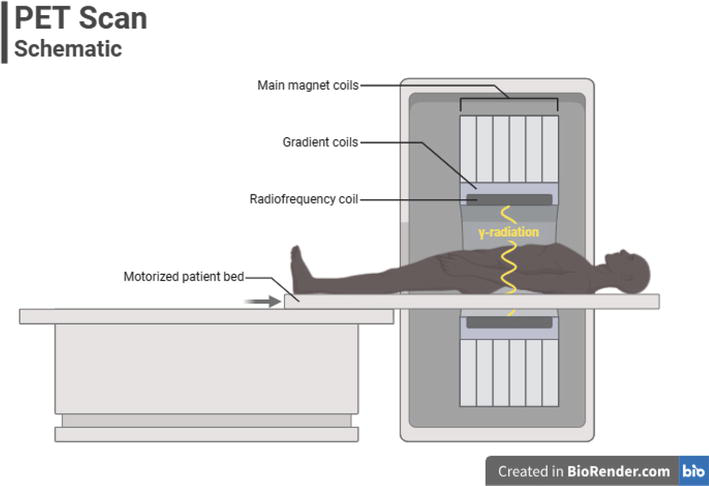
Figure 1.
PET scan schematic.
Positron emission tomography (PET) scans are of significant importance in the field of cancer due to their ability to provide insights into the metabolic activity of cells. Cancer cells exhibit enhanced metabolic activity, resulting in increased uptake of the radioactive tracer and thus manifesting as heightened brightness on positron emission tomography (PET) pictures. The inclusion of this characteristic makes PET scans a very effective modality for the detection of cancer, evaluation of tumor metastasis, and surveillance of the efficacy of cancer therapies [10]. The use of gamma rays in medicine brings numerous benefits. In treatment, the precision of techniques like Gamma Knife surgery allows for effective treatment of tumors with reduced risk and discomfort compared to traditional surgery. In diagnostics, PET scans provide detailed images that are crucial for early detection and treatment planning [11].
However, the use of gamma rays is not without risks. Due to their high energy and ionizing nature, gamma rays can cause damage to living tissues. This necessitates careful control and minimization of exposure to protect patients and medical staff. The risks are mitigated through precise targeting in treatments and minimal tracer usage in diagnostics, but they remain a critical consideration in the medical use of gamma rays [12].
Gamma rays, with their unique properties, have found a wide range of applications in various industrial and environmental contexts, enhancing safety, efficiency, and quality in these sectors. One of the primary industrial uses of gamma rays is in non-destructive testing (NDT). This process involves inspecting materials, components, or assemblies for defects without causing damage. Gamma rays, due to their high penetrative power, are ideal for this purpose. They can penetrate various materials, including metals, plastics, and ceramics, allowing for the internal examination of objects [13].
In NDT, gamma rays are used to create a radiographic image of the item being inspected. This is similar to taking an X-ray in medical imaging. The gamma rays pass through the object and are captured on a detector or film on the other side [14]. Any flaws in the material, such as cracks, voids, or inclusions, will absorb or block the gamma rays differently than the surrounding material, appearing as distinct anomalies on the radiograph. This method is widely used in various industries, including aerospace, automotive, and construction, to ensure the integrity and safety of components.
Gamma rays are also used in food irradiation, a process to ensure food safety and extend shelf life. Food irradiation involves exposing food to gamma rays to kill bacteria, parasites, and other pathogens, reducing the risk of foodborne illnesses [15]. It also delays the ripening and sprouting in fruits and vegetables, which helps preserve their freshness during transportation and storage.
The process of food irradiation is done using sources like Cobalt-60, which emits gamma rays. The key advantage of using gamma rays is that they effectively sterilize food without raising its temperature, keeping the food fresh and preventing the changes in texture or taste often associated with thermal processing [16].
In environmental monitoring, gamma rays are used to analyze and monitor various environmental parameters. They can be employed in the measurement of soil density and moisture content, which is essential for agriculture and construction projects. Gamma-ray spectrometry is also used to monitor and assess the levels of natural and artificial radionuclides in the environment, an important aspect of environmental safety, especially around nuclear facilities [17].
Furthermore, gamma rays are used in tracking and monitoring pollution. For example, gamma-ray tracing techniques can track the movement of sediments in rivers and oceans, helping in the study of erosion and sedimentation patterns [17].
The use of gamma rays in these applications offers numerous benefits in terms of safety and efficiency. In industrial settings, NDT with gamma rays ensures the safety and reliability of products without damaging them. In food safety, gamma irradiation eliminates harmful organisms, ensuring the safety of the food supply [15]. In environmental monitoring, gamma rays provide precise and non-invasive means of analyzing and tracking various environmental factors.
However, the use of gamma rays also requires strict safety protocols to protect workers and the environment from radiation exposure. Proper shielding, handling, and storage of gamma-ray sources are essential to ensure safety in these applications.
The safe handling and regulation of gamma rays are paramount due to their high energy and potential for biological damage. Understanding and adhering to safety measures and regulatory frameworks are essential to mitigate risks associated with gamma radiation in various applications, from medical treatments to industrial processes [18].
One of the primary safety measures in working with gamma rays is the use of adequate shielding. Materials such as lead, concrete, or specialized alloys are often used to absorb or reduce gamma radiation, thereby protecting personnel and the environment [19].
Minimizing the time spent near gamma radiation sources and maximizing the distance from them are key safety practices. This reduces the exposure and, consequently, the dose of radiation received. In environments where gamma radiation is present, wearing appropriate protective equipment, such as lead aprons or shields, is crucial for minimizing exposure. The use of radiation detectors and dosimeters is essential for monitoring radiation levels and individual exposure. These devices help in ensuring that exposure stays within safe limits [20].
Comprehensive training for personnel working with gamma rays is essential. This includes understanding the properties of gamma radiation, knowing the safety protocols, and being prepared for emergency situations.
The International Atomic Energy Agency (IAEA) and the World Health Organization (WHO) play a pivotal role in outlining guidelines and standards for the safe utilization of gamma rays, especially in medical and industrial contexts. Countries typically have their own regulatory bodies and specific regulations to govern the use of radiation. These national regulations dictate permissible exposure limits, safety protocols, and requirements for obtaining licenses and conducting inspections [21]. Entities employing gamma rays are mandated to acquire the necessary licenses. They are also subject to routine inspections to ensure adherence to safety standards. Additionally, regulations encompass the safe management, storage, and disposal of radioactive materials and waste, which is vital for averting environmental contamination and public exposure.
The responsible handling of gamma rays transcends regulatory compliance; it is a moral obligation to guarantee public and environmental safety. Improper management or negligence in the handling of gamma rays can result in severe health issues like radiation sickness, heightened cancer risks, and environmental damage [22].
In medical settings, gamma rays are indispensable for diagnostics and treatment. However, their application necessitates a delicate equilibrium between therapeutic advantages and potential risks. Practices such as precise targeting in Gamma Knife surgery and minimizing unnecessary exposure in diagnostic processes are crucial [23]. In industrial and environmental sectors, safety protocols are crucial to safeguard workers and the public from unintended exposure. This is especially significant in industries like aerospace and construction, where material integrity is essential for safety.
3. Environmental monitoring
Environmental monitoring plays a crucial role in comprehending and controlling the well-being of our ecosystems. Gamma rays have become a potent means of evaluating and tracking environmental conditions, out of the many tools and approaches used [24]. Gamma rays are a form of electromagnetic radiation with high energy that is emitted from the nuclei of atoms. They possess the ability to permeate through a majority of substances, rendering them valuable in the identification and quantification of diverse chemicals, particularly those that are radioactive [25].
Gamma-ray spectroscopy is employed to cartographically identify both naturally occurring and human-made radioactive substances present in the surroundings. Gamma-ray spectroscopy exploits the phenomenon that distinct radioactive isotopes release distinctive gamma rays at precise energy levels. Through the utilization of detectors capable of correctly quantifying the exact energy of gamma rays released from a specific place, scientists can determine the types and quantities of radioactive elements present [26].
Gamma spectroscopy can be employed in aerial radiation mapping studies to detect and identify both naturally occurring and artificially generated radioisotopes in the environment. Helicopters and airplanes fitted with gamma spectrometers conduct aerial surveys in a systematic pattern over a region, collecting data that is combined with GPS information to generate intricate maps illustrating radiation levels throughout the terrain [27]. These maps have the ability to identify higher levels of uranium, thorium, potassium-40, or other radioactive elements that are linked to specific rocks or minerals.
Gamma spectroscopic mapping allows for the detection and monitoring of man-made radioactive pollution resulting from nuclear accidents, fallout from weapons testing, and incorrect disposal of radioactive waste, among other sources. The Chernobyl tragedy, for example, enabled scientists to comprehensively determine the extent to which radiation spread. Following the Fukushima nuclear accident, highly sensitive equipment was used to identify even small amounts of pollution on the opposite side of the Pacific Ocean [28].
Gamma spectroscopy mapping data provides accurate radiological profiles of landscapes and exact distribution patterns of isotopes. This information enables authorities to enhance their monitoring of environmental radioactivity, evaluate hazards, respond effectively to emergencies, find misplaced radioactive sources, and do other related tasks.
Gamma-ray equipment is employed to monitor post-nuclear incident environments and evaluate the dispersion and density of radioactive pollutants. Following an accidental or intentional nuclear release, it is crucial to promptly assess the extent and scale of radioactive contamination in the affected regions. Utilizing gamma-ray surveying instruments on vehicles, drones, robots, or handled by reaction workers can be highly advantageous for this undertaking [29].
Cesium-137, a type of fission product, emits gamma rays that have the ability to permeate through several commonly found materials. Gamma detectors with high sensitivity and precise directionality, along with global positioning systems, have the capability to monitor contamination levels in real-time. This enables the identification and mapping of dangers with precise geographical accuracy, which may then be used to inform decisions on evacuation and remediation [30]. Utilizing aerial gamma surveys conducted by manned helicopters or drones provides responders with rapid acquisition of high-resolution data, especially in cases where infrastructure has been significantly compromised.
Both air sampling using particulate filters and ground-level gamma scans are essential for comprehensive environmental monitoring. Isotope identities provide confirmation of the specific nuclear materials that were released, which is crucial for supporting the medical treatment of those who have been exposed if such treatment is available. Conducting multiple surveys enables the measurement of changes in radioactive decay and contaminant movement over a period of time [31].
State-of-the-art spectroscopic gamma detectors offer the highest level of accuracy in measurements. When used in conjunction with suitable modeling approaches, this technology has the capability to produce 3D contamination maps that provide estimates of material depth and activity levels [32]. Ensuring high-quality monitoring after an incident is crucial for effectively controlling health risks and providing guidance for intricate, prolonged remediation processes.
Gamma rays are used to ascertain the concentration of natural radionuclides in soils, a vital factor in comprehending soil health and fertility. The concentration of natural radioisotopes in soil is directly associated with important indices of soil quality, such as mineral content, texture, and cation exchange capacity (CEC) - which refers to the soil’s ability to retain nutrients [33]. Gamma-ray spectroscopy enables the precise quantification of radioisotopes such as potassium-40, uranium-238, thorium-232, and radioactive cesium, which are commonly found in clay and organic materials.
Utilizing airborne gamma-ray sensors to survey extensive agricultural regions can effectively delineate the fluctuation in these radioisotopes. By utilizing this method, it becomes possible to draw conclusions regarding the distribution of soil composition zones and the variations in fertility levels throughout extensive territories [34]. This aids in the identification of regions with lower nutrient levels or water retention capacities, therefore providing valuable insights for agricultural management strategies.
Portable gamma-ray spectrometers that are located on the ground can also investigate the concentrations of radioisotopes. The examination of paired soil samples in a laboratory improves the understanding of the relationships between radioisotope signals, underlying geology, and agricultural qualities such as permeability, porosity, drainage class, and particle size distribution [34]. This data enhances comprehension of soil quality at smaller, more specific scales.
Gamma rays possess non-invasive properties and exceptional penetration capabilities, rendering them an exceptionally valuable tool for deducing both physical and chemical attributes of soil. Gamma scanning, when used in soil genesis studies, can reveal regional variations in weathering and geochemical history [35]. These insights can assist in directing the process of restoring soils that have been damaged.
The utilization of gamma-ray monitoring is implemented to identify and measure radionuclides in bodies of water, guaranteeing the security of potable water and aquatic ecosystems. In response to the Fukushima nuclear accident, authorities in the United States and Canada established coastal monitoring programs utilizing gamma spectrometry to monitor the dispersion of cesium, iodine, and other radioactive isotopes carried over the Pacific Ocean and into coastal waterways [36]. This facilitated the prompt identification of any potential contamination hazards. Gamma dose rate meters installed on floating devices and buoys, which are linked to wireless networks, have the capability to offer immediate and continuous monitoring of radiation levels in reservoir water. They can even detect sudden releases of radiation from nuclear reactors [37].
Drinking water quality can be affected by the presence of natural radioisotopes in aquifer rock and sediments. In a study conducted in 2022, gamma spectroscopy was employed to examine the levels of radium in water distribution infrastructure. This analysis helped to identify specific places and mechanisms that contribute to higher levels of radioactivity in consumer tap water [38]. Researchers are currently working on the development of portable gamma detectors for quick and cost-effective monitoring of water produced from fracking operations. These detectors will be used with lab-based ICP-MS analysis to detect short half-life radionuclides.
Gamma-ray analysis has the capability to identify and measure radionuclides present in the air, which serve as reliable markers of both pollution and nuclear activity. Natural radionuclides such as radon-222, as well as manufactured isotopes, have the potential to accumulate in the surrounding air, which can be harmful if inhaled. According to Shakhashiro et al. [39], gamma spectroscopy is the most sensitive method for detecting and determining the exact composition of radioactive substances in the air.
Networks of air particle monitoring stations equipped with gamma detectors provide uninterrupted data on ambient radiation levels and radioactive concentrations. It is necessary to investigate any abnormal increases in order to identify potential sources of pollution or radioactive releases before the general population is exposed to them [40]. The ability to be aware of events as they happen in real time enables authorities to make timely and effective decisions.
Various incident scenarios can result in the release of gamma-emitting radioisotopes into the atmosphere. These encompass incidents at nuclear sites, mishandling of medicinal or industrial isotopes, detonation of radiological dispersal devices, and the dissemination of fallout particles that have been stirred up again. Identifying distinct fission product signals aids in determining the origin [41].
Drones and unmanned robots, which are equipped with small gamma spectrometers, provide flexibility in mapping incidents from the air and ground, while also lowering the amount of radiation exposure for responders [42]. Understanding the dispersal of pollution is crucial for both protection and repair efforts.
Scientists employ gamma rays to investigate the effects of radiation on flora and fauna, thereby aiding in the preservation of biodiversity. Living beings can be affected by gamma rays through many methods, which result in the transfer of energy that can modify cell function and DNA. The process of quantifying radiation effects aids in the prediction of ecosystem stability and the establishment of safety limits for animals concerning contamination events or prolonged exposure [43].
Field gamma spectrometry surveys assess the radiation levels in both natural background and elevated environments by mapping the distribution of radionuclides in soil and food chains. The study conducted by Nikolova et al. [44] demonstrates the use of paired biological sampling to investigate the dynamics of radioisotope transfer and to establish benchmarks for biodiversity.
The study conducted by Thiemann et al. [45] involves analyzing the genomes, physiology, and morphology of animals from multiple generations that have been exposed to controlled gamma, x-ray, or ion beam irradiation. The purpose of this study is to understand the rates of mutations and the mechanisms of adaption. This demonstrates the diversity in resistance to radiation among different species.
Some plant studies have shown that exposure to radiation can increase genetic variety, which may help protect ecosystems against environmental changes at low doses [46]. Nevertheless, some studies have observed reduced germination, slowed growth, and lethal effects beyond specific dosage thresholds [47].
Gamma-ray spectroscopy is an advanced analytical method employed to detect and measure radionuclides in samples from the environment. Through the examination of gamma-ray spectra, which represent the dispersion of gamma-ray energy released by radioactive substances, this technique may precisely ascertain the specific types and quantities of radionuclides that are present [48]. Mastering this method is crucial for comprehending ambient radioactivity, particularly in regions impacted by nuclear operations or mishaps. The method’s precision and dependability establish it as a fundamental technique in the monitoring of environmental radiation. Accurate interpretation and analysis of gamma-ray spectra necessitate a specialist understanding of nuclear physics due to their intricate complexity.
Portable gamma-ray detectors are essential in field studies. They enable the collection of measurements directly at the location and prompt examination of environmental samples [49]. These instruments are highly valuable for quickly evaluating radiation levels in many contexts, ranging from natural landscapes to metropolitan surroundings. Their portability allows researchers and workers to carry out surveys in regions where laboratory analysis may be impractical or unfeasible. Although these detectors are highly adaptable, they frequently encounter constraints in terms of sensitivity and accuracy when compared to more advanced laboratory equipment [49].
Satellite and aerial-based gamma-ray sensors enable remote sensing, providing the ability to map broad areas for environmental monitoring purposes. This technology is crucial for conducting large-scale surveys, enabling the identification and cartography of radioactive substances across wide areas [50]. It is highly efficient in locations that are difficult to reach or pose risks, as it can gather crucial data without requiring the collection of physical samples. These technologies play a crucial role in conducting extensive environmental evaluations, particularly in monitoring the spread of radioactive pollutants after a nuclear incident [51].
Although these technologies provide substantial advantages, their deployment involves several problems and concerns. Deciphering gamma-ray data is intricate and necessitates a profound comprehension of nuclear physics and environmental science. The complexity of the data necessitates careful analysis, as any misinterpretation can result in erroneous conclusions regarding environmental conditions. The sensitivity and precision of gamma-ray devices, particularly portable detectors, can occasionally restrict the range of detection and analysis. This constraint is a pivotal element for carrying out intricate investigations or handling radiation at a minimal intensity [29]. Strict compliance with safety regulations and regulatory criteria is necessary while using gamma-ray equipment. Comprehensive safety procedures are required while handling radioactive materials and operating equipment that emits gamma rays in order to ensure the protection of both operators and the environment.
4. Expanding applications and future potential
Gamma rays possess distinctive properties including penetration, sterilization, and material activation capabilities that researchers continue finding innovative applications for across scientific and industrial fields.
Gamma radiography gauges enable non-destructive analysis of density variances and physical integrity in materials from concrete to aerospace composites [52].
Food and polymer irradiation harnesses high gamma energy to alter chemical or molecular structures, creating improved material properties or killing pathogens [53].
Emerging x-ray fluorescence and gamma-ray computed tomography scans paired with carbon dating verify the age, origins, and preservation states of rare organic items [54].
Gamma-ray scanning portals provide drive-through screening of shipping containers, catching shielded nuclear materials [55]. Detection networks leverage signals from fission events for tracking weapons tests and detonations with high accuracy. 3D gamma spectroscopy mapping of nuclear forensics debris pinpoints device attributes aiding attribution [56]. Lab demonstrations of modulated gamma-ray optical communication signals exhibit resistance to scattering with potential for ultra-secure space transmissions [57]. The versatility and value proposition of gamma radiation will continue to extend across various applications in the future decades as advancements in source, sensor, compute, and data fusion technologies evolve [58]. The immense energy of gamma rays could potentially be utilized in the realm of quantum computing and communication. While this use is more speculative, the possibility of using gamma rays to manipulate quantum states has the potential to create new opportunities in this advanced subject [58]. Investigating the capacity of gamma rays for energy generation, specifically in domains such as nuclear fusion, holds promise as a prospective field of study. Although it presents difficulties, utilizing gamma rays for energy has the potential to offer a novel approach to clean and efficient power production [59]. With the progression of technology, the possibilities for using gamma rays are expected to broaden, presenting inventive solutions and unveiling novel opportunities in the fields of research and technology.
References
- 1.
Philippov A, Kramer M. Pulsar magnetospheres and their radiation. Annual Review of Astronomy and Astrophysics. 2022; 60 :495-558 - 2.
Gupta TK, Gupta TK. Nuclear radiation, ionization, and radioactivity. In: Radiation, Ionization, and Detection in Nuclear Medicine. United States: Springer; 2013. pp. 1-57 - 3.
Vallabhajosula S. Radioactivity. In: Molecular imaging and targeted therapy: Radiopharmaceuticals and clinical applications. United States: Springer; 2023. pp. 49-62 - 4.
Diehl R, Korn AJ, Leibundgut B, Lugaro M, Wallner A. Cosmic nucleosynthesis: A multi-messenger challenge. Progress in Particle and Nuclear Physics. Vol. 127. Amsterdam, Netherlands: Elsevier; 2022. pp. 103983 - 5.
Persson BRR. Radiation hazards. In: Occupational Hazards in the Health Professions. CRC Press; 2020. pp. 163-235 - 6.
Giommi P, Padovani P, Polenta G. A simplified view of blazars: The γ-ray case. Monthly Notices of the Royal Astronomical Society. Oxford University. 2013; 431 (2):1914-1922 - 7.
Lindquist C. Gamma knife radiosurgery. In: Seminars in Radiation Oncology. Amsterdam: Elsevier; 1995. pp. 197-202 - 8.
Niranjan A, Bowden G, Flickinger JC, Lunsford LD. Gamma knife radiosurgery. Principles and Practice of Stereotactic Radiosurgery. United States: Springer; 2015. pp. 111-119 - 9.
Terziċ A, Goerres G. Diagnostic imaging–positron emission tomography, combined PET/CT imaging. In: Osteomyelitis of the Jaws. United States: Springer; 2008. pp. 113-119 - 10.
Gallamini A, Zwarthoed C, Borra A. Positron emission tomography (PET) in oncology. Cancers (Basel). 2014; 6 :1821-1889 - 11.
Reynaert N. PET and MRI based RT treatment planning: Handling uncertainties. Cancer/Radiothérapie. 2019; 23 :753-760 - 12.
Harrell CR, Djonov V, Fellabaum C, Volarevic V. Risks of using sterilization by gamma radiation: The other side of the coin. International Journal of Medical Sciences. 2018; 15 :274 - 13.
Wang B, Zhong S, Lee T-L, Fancey KS, Mi J. Non-destructive testing and evaluation of composite materials/structures: A state-of-the-art review. Advances in Mechanical Engineering. 2020; 12 :1687814020913761 - 14.
Rapaport MS, Gayer A. Application of gamma ray computed tomography to nondestructive testing. NDT E International. 1991; 24 :141-144 - 15.
Indiarto R, Pratama AW, Sari TI, Theodora HC. Food irradiation technology: A review of the uses and their capabilities. International Journal of Engineering Trends and Technology (IJETT). 2020; 68 :91-98 - 16.
Ashraf S, Sood M, Bandral JD, Trilokia M, Manzoor M. Food irradiation: A review. International Journal of Chemical Studies. 2019; 7 :131-136 - 17.
Goldsten JO, Rhodes EA, Boynton WV, Feldman WC, Lawrence DJ, Trombka JI, et al. The MESSENGER gamma-ray and neutron spectrometer. The Messenger Mission to Mercury. United States: Springer; 2007. pp. 339-391 - 18.
Sarkar PS. Safety, regulations, metrology and standards in neutron imaging. In: Neutron Imaging: Basics, Techniques and Applications. Germany: Springer; 2022. pp. 207-235 - 19.
Kaur T, Sharma J, Singh T. Review on scope of metallic alloys in gamma rays shield designing. Progress in Nuclear Energy. 2019; 113 :95-113 - 20.
NG SE, SA F. Assessment of awareness and practice of ionizing radiation protection procedures among exposed health care workers. Egyptian Journal of Occupational Medicine. 2020; 44 :529-544 - 21.
Anurag L, Gaurav M, Sanjay D, Chandrashekar M. Spectrum of IAEA standard with regard to radiation application. Journal of Datta Meghe Institute of Medical Sciences University. 2022; 17 :461-467 - 22.
Avendaño G. Critical importance of multilateral studies related with adverse events in medical devices. Health and Technology (Berl). 2016; 6 :213-227 - 23.
Luharia A, Mishra G, Saroj D, Sonwani V, Dhoble SJ. The role of physics in modern radiotherapy: Current advances and developments. Photophysics and Nanophysics in Therapeutics. USA: Elsevier; 2022. pp. 139-162 - 24.
Rodrigues EA, Ferreira ML, de Carvalho AR, Bustillos JOWV, Victor RABM, Sodré MG, et al. Land, water, and climate issues in large and megacities under the lens of nuclear science: An approach for achieving sustainable development goal (SDG11). Sustainability. 2022; 14 :13646 - 25.
Mosayebnia M, Ahmadi M, Emzhik M, Hajiramezanali M. Gamma-ray involved in cancer therapy and imaging. In: Electromagnetic Waves-Based Cancer Diagnosis and Therapy. Amsterdam, Netherlands: Elsevier; 2023. pp. 295-345 - 26.
El-Taher A. Nuclear analytical techniques for detection of rare earth elements. International Journal of Radiation Applications and Instrumentation. 2018; 3 :53-64 - 27.
Varley A, Tyler A, Smith L, Dale P, Davies M. Mapping the spatial distribution and activity of 226Ra at legacy sites through Machine Learning interpretation of gamma-ray spectrometry data. Science of the Total Environment. 2016; 545 :654-661 - 28.
Buesseler K, Dai M, Aoyama M, Benitez-Nelson C, Charmasson S, Higley K, et al. Fukushima Daiichi–derived radionuclides in the ocean: transport, fate, and impacts. Annual Review of Marine Science. 2017; 9 :173-203 - 29.
Marques L, Vale A, Vaz P. State-of-the-art mobile radiation detection systems for different scenarios. Sensors. 2021; 21 :1051 - 30.
Randall GL, Iglesis E, Wong HF, Speller RS. A method of providing directionality for ionising radiation detectors—RadICAL. Journal of Instrumentation. 2014; 9 :P10011 - 31.
Chartin C, Evrard O, Onda Y, Patin J, Lefèvre I, Ottlé C, et al. Tracking the early dispersion of contaminated sediment along rivers draining the Fukushima radioactive pollution plume. Anthropocene. 2013; 1 :23-34 - 32.
Verbelen Y, Martin PG, Ahmad K, Kaluvan S, Scott TB. Miniaturised low-cost gamma scanning platform for contamination identification, localisation and characterisation: A new instrument in the decommissioning toolkit. Sensors. 2021; 21 :2884 - 33.
Silvero NEQ , Demattê JAM, Minasny B, Rosin NA, Nascimento JG, Albarracín HSR, et al. Sensing technologies for characterizing and monitoring soil functions: A review. Advances in Agronomy. 2023; 177 :125-168 - 34.
Moonjun R, Shrestha DP, Jetten VG, van Ruitenbeek FJA. Application of airborne gamma-ray imagery to assist soil survey: A case study from Thailand. Geoderma. 2017; 289 :196-212 - 35.
Guagliardi I, Rovella N, Apollaro C, Bloise A, De Rosa R, Scarciglia F, et al. Effects of source rocks, soil features and climate on natural gamma radioactivity in the Crati valley (Calabria, Southern Italy). Chemosphere. 2016; 150 :97-108 - 36.
Cooke MW, Trudel M, Gurney-Smith HJ, Kellogg JP, Cullen JT, Francisco BBA, et al. Radioactivity concentration measurements in fish and shellfish samples from the west coast of Canada after the Fukushima nuclear accident (2011-2018). Journal of Environmental Radioactivity. 2022; 251 :106934 - 37.
Arndt J, Kirchner JS, Jewell KS, Schluesener MP, Wick A, Ternes TA, et al. Making waves: Time for chemical surface water quality monitoring to catch up with its technical potential. Water Research. 2022; 213 :118168 - 38.
Vengosh A, Coyte RM, Podgorski J, Johnson TM. A critical review on the occurrence and distribution of the uranium-and thorium-decay nuclides and their effect on the quality of groundwater. Science of the Total Environment. 2022; 808 :151914 - 39.
Shakhashiro A, Doherty P, Logar JK, Vodenik B, Verheyen L, Taggart M. New certified reference materials and proficiency test for environmental radioactivity measurements. Accreditation and Quality Assurance. 2016; 21 :351-360 - 40.
Barescut JC, Gariel JC, Péres JM, Balonov M, Linsley G, Louvat D, et al. The IAEA standards for the radioactive discharge control: Present status and future development. Radioprotection. 2005; 40 :S721-S726 - 41.
Vilardi I, Antonacci G, Battisti P, Castellani CM, Ciciani L, Del Gaudio D, et al. Large-scale individual monitoring of internal contamination by gamma-emitting radionuclides in nuclear accident scenarios. Journal of Radiological Protection. 2019; 40 :134 - 42.
Martin PG, Kwong S, Smith NT, Yamashiki Y, Payton OD, Russell-Pavier FS, et al. 3D unmanned aerial vehicle radiation mapping for assessing contaminant distribution and mobility. International Journal of Applied Earth Observation and Geoinformation. Vol. 52. Amsterdam, Netherlands: Elsevier; 2016. pp. 12-19 - 43.
Agency IAE. Effects of ionizing radiation on plants and animals at levels implied by current radiation protection standards. IAEA; 1992 - 44.
Nikolova I, Johanson KJ, Clegg S. The accumulation of 137Cs in the biological compartment of forest soils. Journal of Environmental Radioactivity. 2000; 47 :319-326 - 45.
Thiemann RA, Thornton JK, Stayer PA, Riley E, Clark R, Armour N, et al. A Novel presentation of clostridium perfringens in young broilers. Avian Diseases. 2022; 66 :205-212 - 46.
Maity TN, Saha AK, Dubey A, Laha R. Search for dark matter using sub-PeV γ-rays observed by Tibet AS γ. Physical Review D. 2022; 105 :L041301 - 47.
Tran TLC, Callahan DL, Islam MT, Wang Y, Arioli T, Cahill D. Comparative metabolomic profiling of Arabidopsis thaliana roots and leaves reveals complex response mechanisms induced by a seaweed extract. Frontiers in Plant Science. 2023; 14 :1114172 - 48.
Mahmood HS, Hoogmoed WB, Van Henten EJ. Proximal gamma-ray spectroscopy to predict soil properties using windows and full-spectrum analysis methods. Sensors. 2013; 13 :16263-16280 - 49.
Al Hamrashdi H, Cheneler D, Monk SD. A fast and portable imager for neutron and gamma emitting radionuclides. Nuclear Instruments and Methods in Physics Research Section A: Accelerators, Spectrometers, Detectors and Associated Equipment. 2020; 953 :163253 - 50.
Zhang J, Huang Y, Pu R, Gonzalez-Moreno P, Yuan L, Wu K, et al. Monitoring plant diseases and pests through remote sensing technology: A review. Computers and Electronics in Agriculture. 2019; 165 :104943 - 51.
Abdulraheem MI, Zhang W, Li S, Moshayedi AJ, Farooque AA, Hu J. Advancement of remote sensing for soil measurements and applications: A comprehensive review. Sustainability. 2023; 15 :15444 - 52.
Groysman A. Nondestructive testing and corrosion monitoring. Non-Destructive Evaluation of Corrosion and Corrosion-assisted Cracking. Hoboken, New Jersey, U.S: Wiley; 2019. pp. 261-409 - 53.
Farkas J, Mohácsi-Farkas C. History and future of food irradiation. Trends in Food Science and Technology. 2011; 22 :121-126 - 54.
Miyahara AAL, Paixão CP, dos Santos DR, Pagin-Cláudio F, da Silva GJ, Bertoleti IAF, et al. Urban dendrochronology toolkit for evidence-based decision-making on climate risk, cultural heritage, environmental pollution, and tree management–A systematic review. Environmental Science & Policy. 2022; 137 :152-163 - 55.
Shakhashiro A, Sansone U, Wershofen H, Bollhöfer A, Kim CK, Kim CS, et al. The new IAEA reference material: IAEA-434 technologically enhanced naturally occurring radioactive materials (TENORM) in phosphogypsum. Applied Radiation and Isotopes. 2011; 69 :231-236 - 56.
Varga Z, Wallenius M, Krachler M, Rauff-Nisthar N, Fongaro L, Knott A, et al. Trends and perspectives in nuclear forensic science. TrAC Trends in Analytical Chemistry. 2022; 146 :116503 - 57.
De Angelis A, Tatischeff V, Argan A, Brandt S, Bulgarelli A, Bykov A, et al. Gamma-ray astrophysics in the MeV range: The ASTROGAM concept and beyond. Experimental Astronomy. 2021; 51 :1225-1254 - 58.
Wright T, West A, Licata M, Hawes N, Lennox B. Simulating ionising radiation in gazebo for robotic nuclear inspection challenges. Robotics. 2021; 10 :86 - 59.
Howell CR, Ahmed MW, Afanasev A, Alesini D, Annand JRM, Aprahamian A, et al. International workshop on next generation gamma-ray source. Journal of Physics. G, Nuclear and Particle Physics: An Institute of Physics Journal. 2021; 49 :10502