ASTM standards about coating durability.
Abstract
Icing is a severe hazard to the flight safety of unmanned aerial vehicles (UAV) in cold climates. Great efforts have been put into developing a reliable and practical anti-/de-icing system for icing mitigation. Compared to the conventional thermal-based deicing systems, applying superhydrophobic coatings with excellent ice-repellent performance is a promising anti-icing technique with low weight and low energy input. However, the application of superhydrophobic coatings on UAV is hindered by the substandard durability. This chapter comprehensively reviews advances in durability tests of superhydrophobic coatings pertinent to UAV anti-/de-icing. This chapter specifically introduces the mechanism of degrading superhydrophobic coatings by droplet erosion, which is rarely discussed in previous studies but can cause severe damage to the surface topology by applying “water hammer pressure.” Consequently, recommendations are provided to facilitate a more systematic approach to conducting durability tests for superhydrophobic coatings.
Keywords
- durability
- superhydrophobic coating
- anti-icing
- droplet erosion
- aircraft icing
1. Introduction
Aircraft icing plays a critical factor in endangering flight safety, since it can distort airflow over the wing, degrade the controllability, cause the stall at a lower angle of attack and lead to roll and pitch upsets [1]. Federal Aviation Administration (FAA), European Union Aviation Safety Agency and Civil Aviation Administration of China have issued strict icing airworthiness regulations because in-flight icing has resulted in several severe aviation accidents [2]. For example, American Eagle Flight 4184 crashed because of icing, resulting in 68 fatalities; and in 2004, China Eastern Airlines Flight MU5210 crashed due to wing icing (or frost), resulting in the loss of 55 lives. Data disclosed by FAA show that icing incidents led to 46 flight safety accidents between 2008 and 2016 [3]. The development of unmanned aerial vehicles (UAV) has indeed seen rapid growth in recent years, which is attributed to the increasing demand in the civil and military applications. However, during military operations, 25% of UAV flights encountered icing, which had a detrimental impact on mission success [4]. Common strategies for UAV icing avoidance involve either grounding the UAV or modifying path planning, which significantly reduces the operational capabilities of the UAV in cold climates [5].
Advanced anti-/de-icing systems are essential protections for aircraft in adverse weather conditions. Most airliners rely on the thermal deicing system, which involves heating the icing components during flight to remove the accreted ice. The thermal de-icing method uses either hot air from the engine or electrical heating parts to melt the accreted ice [6]. High-voltage plasma deicing is also a promising thermal deicing method, which can achieve the flow and icing control with the same equipment [7]. However, these anti-/de-icing techniques may be too complex, heavy and power-intensive to be practical for UAV, and therefore, may not be suitable for small-scale, lightweight UAV due to payload limitations and limited available power [8]. As a result, a hybrid deicing system combining a thermal deicing system on the wing/propeller’s leading edge and a superhydrophobic coating covering the wing surface was developed as a low-power hybrid deicing strategy [9]. This hybrid deicing method has been used on unmanned aviation vehicles and wind turbines. As a part of the hybrid deicing system, superhydrophobic coating is a promising anti-icing technology with lightweight, low energy consumption and excellent water/ice repellency [10]. Because of the air pockets underneath the water droplet or ice, some superhydrophobic coatings can significantly reduce ice adhesion by reducing surface energy and delaying icing time. Microcraks between the solid surface and the water or ice may be introduced by the air pockets. The size of the microcracks is the key factor that governs ice adhesion. Consequently, some superhydrophobic surfaces significantly reduce ice adhesion if they provide sufficiently large microcraks at the interface.
Superhydrophobic coatings can be fabricated in many ways, but the primary approach is either patterning roughness on the solid surface, followed by attaching a thin layer of a low surface energy material or depositing materials with nanostructures and low surface energy on the surface. Fluoropolymers or siloxanes are classical materials with low surface energy employed to achieve superhydrophobicity [11]. Common techniques to fabricate superhydrophobic coatings include lithography, sol-gel method, electrochemical deposition, chemical vapor deposition, spraying, imprinting, hydrothermal method and phase separation [12]. Yang et al. [13] reported a facile phase separation route to produce anti-corrosion superhydrophobic polyvinyl-chloride coatings on a magnesium alloy (see Figure 1(a)). Ding et al. [14] described the construction of the superhydrophobic surface by electrospinning the poly(vinyl alcohol) and poly(vinyl alcohol)/zinc acetate solutions followed by the calcination process, which yields fibrous zinc oxide superhydrophobic films (see Figure 1(b)). The wetting properties of the fibrous films were further altered by applying a coating of a low surface energy fluoroalkylsilane using hexane. Figure 1(c) shows the schematic diagram of the reproduction route to create a robust superhydrophobic coating using the nanoimprint technique for rose petals [15]. Singh et al. [17] reported the synthesis of superhydrophobic coating on cotton fabrics using a sol-gel route (see Figure 1(d)). In addition to using a single method, Wang et al. [18] obtained a highly durable superhydrophobic coating by combining lithography and spraying techniques. Several bulk materials can also provide superhydrophobic surfaces. Zhu et al. [16] developed a superhydrophobic bulk material by embossing carbon nanotubes on polytetrafluoroethylene mold to create the necessary hierarchical texture required for the superhydrophobicity (see Figure 1(e)). Some hydrophilic materials can also be used to fabricate superhydrophobic coatings. Li et al. [19] employed plasma etching and plasma deposition of hydrophilic diamond-like carbon to a paper and successfully got a superhydrophobic surface. The mechanism is that the hierarchical nanostructure of the carbon provides an appropriate roughness to reduce the surface energy.
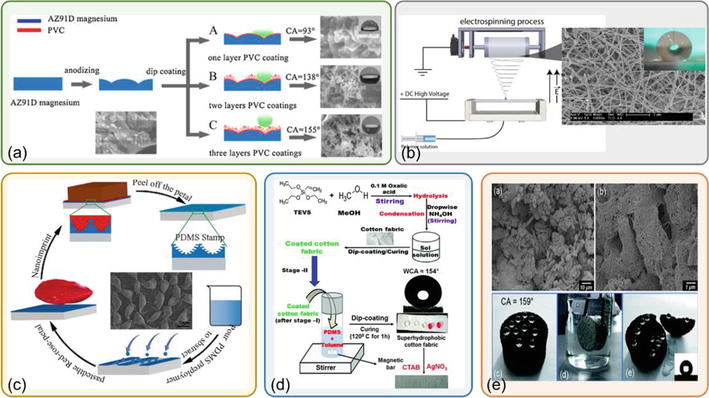
Figure 1.
Techniques to fabricate superhydrophobic coatings: (a) phase separation method, (b) electro-spinning method, (c) nano-imprinting method, (d) hydrothermal method and (e) a superhydrophobic bulk material [
While some superhydrophobic coatings that can provide large microcraks have excellent icephobic performance, none can be used in anti-/de-icing systems of aircraft due to the limited durability. As early as 1969, the National Aeronautics and Space Administration (NASA) collaborated with the FAA to test 100 icephobic coatings in the icing wind tunnel at Glenn Research Center, but none could be used for aircraft applications [20]. NASA then established Revolutionary Icing Materials Evaluation Laboratory (RIMELab, previously referred to as Fundamental Adhesion and Shedding Test laboratory) at the Glenn Research Center, which is equipped with advanced ice adhesion measurement and research equipment. RIMELab is dedicated to developing low-ice-adhesion coatings, testing coating durability and quantifying the ice adhesion strength.
In this way, enhancing the durability of superhydrophobic coatings is crucial for application in aircraft. NASA also highlighted that the development of superhydro-/ice-phobic coatings is mainly hindered by the coating durability subject to the operational environment [21]. As an aeronautic superhydrophobic coating, it should perform well in environments with high humidity, high velocity, low temperature, thermal shocks, mechanical wear, corrosion, intense radiation and high-speed water droplet impacts [22]. As a result, the primary types of durability performance of superhydrophobic coatings are droplet/particle erosion resistance, mechanical abrasion resistance, icing/deicing cycles durability, UV resistance and corrosion resistance [23]. Droplet erosion tests (see Figure 2(a)) simulate the impact of micro-droplets or rain droplets, which could gradually degrade the wettability of superhydrophobic coatings due to a Cassie to Wenzel transition. Droplet erosion could be as destructive as solid particle erosion because of the “water hammer effect” [26, 30]. The water hammer effect in droplet impact refers to the phenomenon where a sudden increase in pressure occurs upon the impact of a droplet on a surface. This effect is caused by the rapid deceleration of the droplet upon impact, leading to a sharp increase in pressure that propagates through the fluid. It can result in the generation of shock waves and high local pressures.
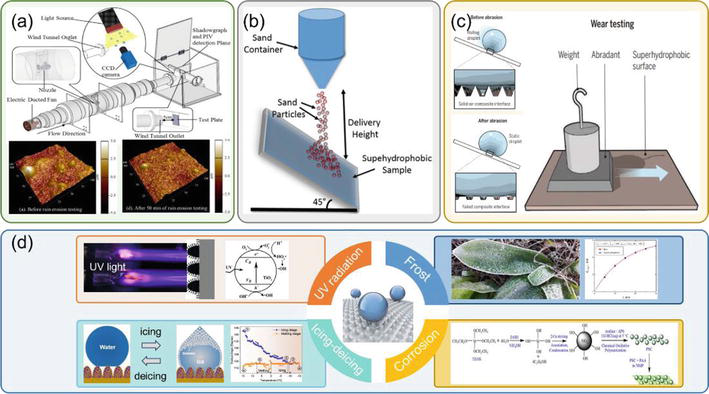
Figure 2.
Classification of the durability of superhydrophobic coatings: (a) droplet erosion test, (b) sand erosion test, (c) mechanical abrasion test, (d) other durability tests [
Particle erosion is a common test performed to evaluate the mechanical durability of superhydrophobic coatings. Figure 2(b) shows a typical experimental setup where particles impinge on the coating with an inclination angle of 45°. These collisions could cause substantial changes to the surface topography, leading to severe destructions of superhydrophobic performance. Mechanical wear is one of the most straightforward and common types of degradation for superhydrophobic coatings; therefore, it is important for characterizing durability [31]. Experiments of mechanical wear have a common concept of rubbing or sliding a solid material into the coating (see Figure 2(c)). Mechanical wear induces material removal that eventually leads to surface topology modification and superhydrophobicity loss. Ultraviolet (UV) light from sunlight could lead to photo-oxidation of superhydrophobic coatings and subsequently the increase of surface energy eventually leads to wettability degradation [32, 33]. Similarly, chemical corrosion, icing-deicing cycle and frost could greatly affect the surface topography and surface energy of superhydrophobic coatings [34].
Plenty of studies have introduced methods of testing or enhancing the durability of superhydrophobic coatings in recent years. However, there is a lack of comprehensive review covering these methods. As a result, this chapter comprehensively summarizes cutting-edge techniques to quantify the surface durability and fabricate durable superhydrophobic coatings. In the following context, the thermodynamics of the surface will be introduced at first to provide the fundamental theory of the wetting phenomenon. Then, methods to quantify the mechanical durability (e.g., sand erosion, droplet erosion and abrasion durability) of superhydrophobic coatings are demonstrated. State-of-art durable coatings that can effectively resist mechanical damage are also introduced. After a comprehensive review of the environmental factors affecting the durability of superhydrophobic coatings, promising approaches to improve the coating durability will be discussed aiming at inspiring great ideas for developing durable, user-friendly and cost-effective superhydrophobic coatings.
2. Thermodynamics of superhydrophobic coatings
Static, receding and advancing contact angles are significant parameters to quantify the wettability of a coating. The contact angle is the angle between the surface tangent on the liquid-vapor interface and the tangent on the solid-liquid interface at their intersection. The static contact angle is the result of the quasi-equilibrium between the liquid, the solid and the vapor phases, so its value on a smooth and homogeneous surface can be given by the Young equation [35]:
where
where
While contact angle can quantify the equilibrium state of droplets, it is not an ideal parameter to predict the friction of droplets. For example, surfaces with “rose-petal-effect” have both high contact angle, high water adhesion and low receding angle, meaning droplets can hardly move on it. As a result, contact angle hysteresis is widely used to quantify the friction of droplets [39, 40]. Contact angle hysteresis (CAH) is defined as the difference between the advancing (
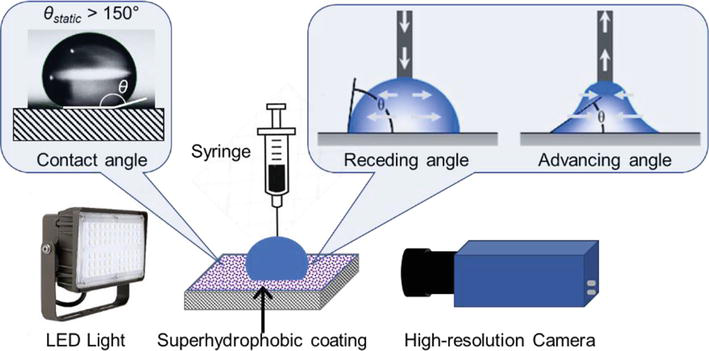
Figure 3.
The experimental setup measures the static, receding and advancing contact angles.
Most superhydrophobic coatings are rough and air gets trapped in their hierarchical structures (named as Cassie-Baxter state), thereby, Eq. (1) needs to be revised as:
where
Recently, the Cassie-Baxter state has been observed in practice by cryo-SEM (see Figure 4) [29, 44]. Figure 4(a) clearly shows that sessile water droplets cannot penetrate the hierarchical structures on the superhydrophobic coating.
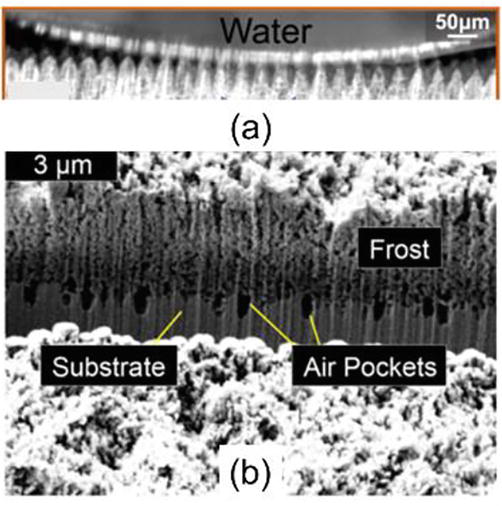
Figure 4.
Experimental evidence of Cassie-Baxter state: (a) a water droplet and (b) a frost layer on a superhydrophobic coating with visible air pockets [
3. Mechanical durability of superhydrophobic coatings
3.1 Droplet erosion
Droplet erosion resistance is one of the most important durability for superhydrophobic coatings pertinent to aircraft/wind turbine icing. Droplet erosion indicates continuous impacts of rain droplets or high-speed airborne droplets [45]. For a representative case of a working offshore wind turbine coated with SHS as an anti-icing coating, rain droplets or sea spray droplets would impinge onto the exposed surface at a high relative velocity of around 80 m/s [46]. Some impinged water droplets may penetrate the micro-scale surface textures of superhydrophobic coatings, which refers to the wetting transition from the non-wetted Cassie-Baxter state to the Wenzel state, thereby, eliminating the hydrophobicity and icephobicity of the SHS. Furthermore, due to the continuous impacts of high-speed droplets, the surface topology of superhydrophobic coatings can be considerably altered and even some materials are worn away from the substrate [26]. It is well known that the superhydrophobicity of a surface is usually achieved by adding hydrophobicity agents onto micro-/nano-textured surfaces [47]. Therefore, superhydrophobic coatings with substantial surface topology change induced by rain erosion may lose the superhydrophobicity.
It should be noted that while extensive studies have been undertaken to investigate droplet erosion effects on different metal surfaces or special erosion-resistant coatings with extremely high-speed drop impacts (i.e., >200 m/s) [48], few studies have discussed the rain erosion mechanisms and effects on the wettability degradation (e.g., increasing contact angle hysteresis) on superhydrophobic coatings. Most of the previous studies about droplet erosion only focused on material or mass loss with extremely high-speed droplet impacts (i.e., >200 m/s). However, droplet-erosion-induced wettability degradation on superhydrophobic coatings occurs with a relatively lower droplet impingement speed (i.e., <100 m/s) without apparent material loss [26]. The water hammer effect in the early stage of the impact was found to be responsible for most of the damage resulting from droplet erosion [49]. In our previous study, we reported the wettability and icephobicity degradation as well as the micro-scale surface topology change upon superhydrophobic coatings after experiencing droplet erosion tests (i.e., impact velocity around 100 m/s), and illustrating the underlying physics of droplet erosion damage by analyzing the fundamentals of the droplet impact on a solid surface [26].
The fundamentals of droplet impact over a solid surface need to be addressed for exploring the mechanisms of rain erosion damage on superhydrophobic coatings. As described by Field [50], the whole impact process of a droplet can be divided into a compressible regime and an incompressible regime based on the progress of shock wave in the droplet. In the compressible regime, Figure 5(a) shows the initial compressible stage where the periphery of the contact area moves outward a short time after impact with a velocity higher than the speed of sound in the liquid and solid. It indicates that a shock wave envelope, after which droplet behaves compressible, is generated immediately giving tremendous pressure to the solid surface. This pressure is usually called “water hammer pressure” whose magnitude can be estimated using Eq. (4) where
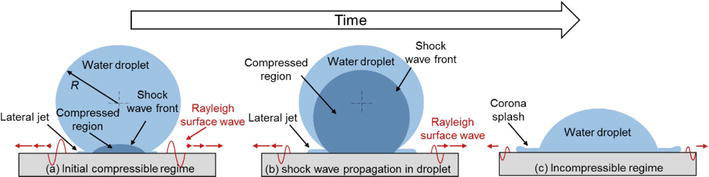
Figure 5.
Different stages of droplet impact on a solid surface. (a). Shock wave generation at the initial compressible regime. (b). Shock wave propagation in the droplet. (c). Incompressible regime.
Eq. (4) is only applied to the ideal solid rigid surface. If the compressibility of the target surface is taken into consideration, the water hammer pressure could be expressed more accurately as:
where
As described by Blower [54] and Athanasopoulos et al. [55], the in-plane amplitude of the Rayleigh surface wave attenuates as it moves away from the impact center (i.e.,
where
All water hammer pressure, dynamic pressure and Rayleigh surface wave are likely to be the main factors causing wettability degradation after rain erosion tests since all of them have different features, which may generate severe damage to superhydrophobic coatings. Although water hammer pressure is tremendous in comparison with the dynamic pressure in terms of stress magnitude, the acting area of it is much smaller than that of the dynamic pressure. For a representative case of a droplet impinging onto an aluminum surface at a velocity of 65 m/s with a diameter of 3 mm, the magnitude of water hammer pressure can reach 89 MPa, significantly higher than only 2 MPa of dynamic pressure. However, the area experiencing the dynamic pressure is 512 times greater than the water hammer pressure. The significantly larger acting area of the dynamic pressure probably leads to more severe damage to the surface since more surface textures in a larger area might be damaged. The amplitude of the Rayleigh surface wave decays with propagating distance, which means the damage caused by the Rayleigh surface wave is higher near the impact center than in the far field. In summary, the importance of these three stresses to the degradation of the SHS due to rain erosion cannot be certainly compared with respect to the stress magnitude or acting area; thereby, the damage contributed by them should be further analyzed and compared considering energy transformation or classical surface fatigue theories.
To protect superhydrophobic coatings, previous studies have been devoted to fabricating durable coatings that resist droplets’ high-speed impact. Brown et al. [56] applied plasma-enhanced chemical vapor deposition to fabricate a new durable superhydrophobic coating, which does not observe failure even after 6000 impacts at 165 m/s (typical speed at the tip of wind turbine blades). Song and Benmeddour [57] developed a new coating comprising a surface-modifying polymer bearing fluorinated and polydimethylsiloxane branches on the erosion-resistant polyurethane matrix to improve the droplet erosion durability. The development of protective coatings to resist droplet erosion should properly use several resins with different properties in combination or modifications to improve the superhydrophobicity as well as the durability [58].
3.2 Sand erosion
Sand erosion is a common and severe wear phenomenon for aircraft and wind turbines in the desert (see Figure 6(a)), which is caused by the repeated impact of sand or other abrasive particles [60]. During operation in desert, forests and near volcanoes, abrasive particles such as sand, dust, coal derivatives and fly ashes impinge on the surface at a high speed (over 100 m/s), leading to material removal and significant changes in the surface topography (see Figure 6(b)). Sand erosion occurs when the abrasive particles strike the coated surface at high velocities, causing the removal or thinning of the coating. While the material removal of ductile materials (e.g., metals and alloys) is caused by the cutting and plowing of the solid particles (see Figure 6(c)), sand erosion leading to crack initiation and propagation (see Figure 6(d)) on brittle materials (e.g., ceramics and silicon wafers) removes material pieces from the surface [61].
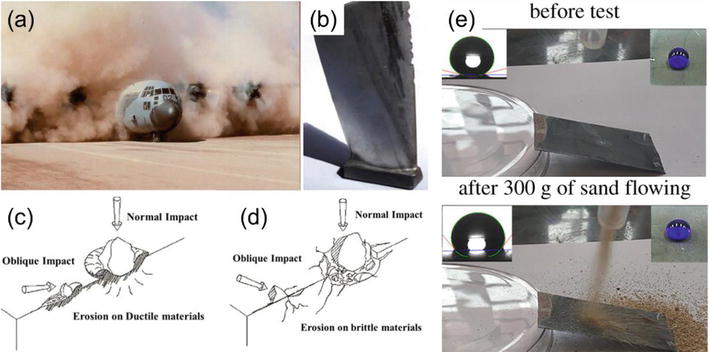
Figure 6.
Sand erosion is a severe problem to (a) aircraft and (b) turbines. The mechanism of sand erosion on (c) ductile and (d) brittle materials [
Many factors influence the material loss rate pertinent to sand erosion such as airflow speed, impact angle, object geometry, surface hardness, fracture toughness and material elasticity. Erosion rate can take the form:
where
Various erosion-resistant coatings for ice protection have been explored in pursuing durable superhydrophobic coatings. Kumar et al. [63] developed a superhydrophobic coating by introducing silica nanoparticles in a polyvinylidene fluoride matrix to improve erosion resistance. The sand erosion test was done by placing the sample surface 10 cm away from the sandblast nozzle at a pressure of 200 kPa for 30 s. After the sand erosion test, the coating with 10% polyvinylidene fluoride remains superhydrophobic. Fu et al. [64] fabricated an erosion-resistant superhydrophobic coating by applying plasma electrolytic oxidation (PEO) and chemical vapor deposition (CVD) on the aluminum substrate. After conducting a sand falling test (i.e., dropping sand particles from a certain height to the surface) using 2000 g sand, the coating remains superhydrophobic. Zheng et al. [65] employed a bio-epoxy resin and SiO2 nanoparticles to make an eco-friendly hydrophobic coating. They found that the coating became superhydrophobic after conducting the sand erosion test since impacts of sand create a porous structure on the surface, allowing more air to be entrapped beneath the droplet.
3.3 Mechanical abrasion
When superhydrophobic coatings are subjected to mechanical abrasion, such as rubbing or scratching, the surface structures can be compromised, significantly degrading the superhydrophobic characteristics. Hensel et al. [66] proposed a method of creating superhydrophobic coatings by mimicking the effectively liquid-repellent and mechanically stable springtail-skin morphology. They tested the durability by oscillating a steel ball with a normal force on the bio-inspired coating. Hensel observed the failure of membranes, which are plastically deformed and delaminated from the substrate, and the pillar arrays that are broken from the bottom (see Figure 7(a)). Kondrashov et al. [67] presented an approach to generate a mechanically robust superhydrophobic coating by growing silicon microcones and nanograss using mask-free dry etching processes. They tested the coating durability by conducting a linear abrasion test, exposing the coating to a normal force and moving the coating back and forth. They found that the coating that combines microcone and nanograss structures (MC-LD) shows greatly improved mechanical durability since microcones can provide an excellent shield to resist wear damage (see Figure 7(b)). MC-LD remains superhydrophobic after the abrasion test with a force of 20 N, but the coating with only nanograss degrades to a hydrophilic coating after experiencing the same test. Dong et al. [68] used the 3D printing technique to fabricate a bulk superhydrophobic material by generating inherently nanoporous structures in the bulk material. In contrast to losing superhydrophobicity after 25 abrasion cycles for those coatings (see Figure 7(c)), the bulk superhydrophobic material keeps low water adhesion and high contact angle after 40 abrasion cycles. Dong’s research shows a promising method to fabricate durable superhydrophobic coatings without concerning the loss of materials.
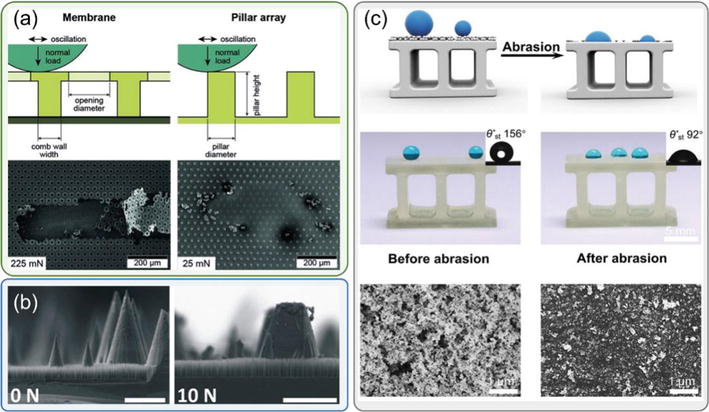
Figure 7.
Mechanical abrasion leads to the compromission of surface topology on superhydrophobic coatings.
The abrasion durability of superhydrophobic coatings can be tested using various methods. The most common method for measuring abrasion resistance involves linear shear abrasion, where a solid abradant moves tangentially across the surface being tested. In this type of wear, the contact point between the surfaces moves parallel to the surface with a force vector perpendicular to the material. Various experimental setups have been used in the literature to study this tangential abrasion, involving the fundamental concept of rubbing or sliding a solid material against the surface under investigation [69, 70]. This process causes mechanical wear, resulting in material removal and eventual alteration of the surface properties, including the loss of non-wettability. Circular and linear abrasion employs similar principles and utilizes the same experimental setup. The main distinction lies in the movement of abradant materials. Circular abrasion involves a disk-shaped abradant material that undergoes rotational motion. In contrast to linear abrasion, one drawback of circular abrasion is the variation in speeds among different sections of the abradant material. As a result, this type of testing is not as commonly used, though the setup is easier to implement. In certain situations, superhydrophobic coatings may encounter sharp objects such as knives, pens, forks, and other items that can cause significant surface damage. Previous studies have conducted blade tests for non-wettable coatings. These tests are essentially similar to linear abrasion tests, but a sharp blade simulates the rubbing action instead of using a flat abradant material.
4. Environmental factors affecting the durability of superhydrophobic coatings pertinent to aircraft/wind turbine icing mitigation
4.1 Icing/deicing cycles
Impact icing and bulk icing are two common icing phenomena related to ice accretion on the surface of aircraft. Impact icing occurs when supercooled large droplets or ice crystals in the atmosphere strike the surface of in-flight aircraft and freeze upon impact. Impact icing typically occurs between 0 and −20°C on unprotected aircraft surfaces, such as the leading edges of wings, engine guide vanes and propellers. It can significantly impact aircraft performance, causing changes in aerodynamics, and impaired control. Bulk icing usually occurs on parked aircraft in freezing drizzles where liquid water freezes on aircraft surfaces without impact or pressure. Bulk icing is smooth and transparent, making it difficult to detect visually.
Since many superhydrophobic coatings have excellent anti-icing performance, such as reducing ice adhesion, delaying freezing time and suppressing ice nucleation temperature, ice adhesion on superhydrophobic coatings is measured in plenty of studies. Bulk and impact ice are two common types of ice used in the measurement. The formation of impact ice is simulating the impact of supercooled droplets or ice crystals onto the aircraft surface using the experimental facility shown in Figure 8(a) [71]. Instead, some previous studies made impact ice by spraying droplets in a cold climate chamber to the surface directly [74]. Since the formation of impact ice involves the impact of droplets, the surface topology of superhydrophobic coatings could be compromised in the same mechanism as the droplet erosion in this process. Besides, before ice nucleation of droplets on the surface, water can infiltrate micro-/nanostructures of superhydrophobic coatings. When water freezes and expands, it can potentially damage or break the surface topology. While impact ice can simulate the in-flight icing process more closely and evaluate the icephobic performance of a coating more accurately, bulk ice is used more frequently due to simple experimental conditions. Figure 8(b) shows a typical facility for icing/deicing cycles that create the bulk ice at first and break the ice sample mechanically then [42]. Another icing/deicing cycle is creating the bulk ice also at first but melting the ice consequently. Although melting bulk ice seems harmless to the superhydrophobic coating, removing the surface material or applying tremendous fracture stress in the icing process could significantly change the surface topology. Mobarakeh and Farzaneh [72] observed that the spongy micro-structures on the surface is replaced by large roughness features after 15 icing/deicing cycles (see Figure 8(c)). Mobarakeh and Farzaneh conduced the icing test at −10°C and the deicing test at the room temperature, which are the icing/deicing conditions used in many literature. Their icing samples were obtained in an icing wind tunnel to simulate the impact icing on UAV. Brown et al. [56] reported that the amount of material is significantly reduced after 80 cycles (See Figure 8(d)). Similarly, Wang et al. [73] proposed that the amount of microcones on the superhydrophobic coating is significantly reduced after 40 melting cycles (See Figure 8(e)). They also conducted the ice-breaking tests using the facility shown in Figure 8(b), and surprising found that 20 breaking cycles contribute less damage to the superhydrophobic coating than 40 melting cycles in terms of surface roughness and microcone number. Their study indicates that the water freezing process severely damages the hierarchical structures more than removing ice from the surface.
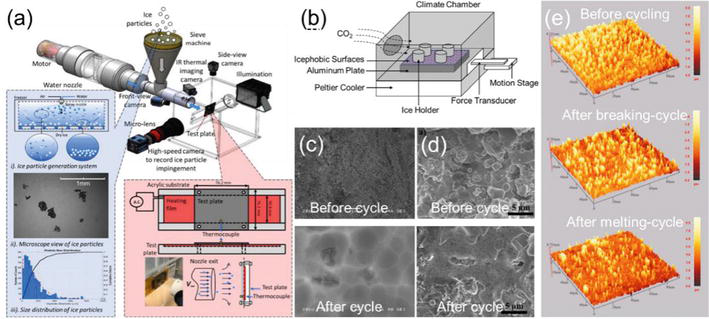
Figure 8.
The experimental setup of creating (a) impact ice samples [
4.2 Chemical corrosion
Corrosion is usually an undesirable and unsecured phenomenon since it has a detrimental impact on the functional characteristics of metals. The total annual cost of corrosion in the petroleum industry of USA is estimated to be 1.4 billion dollars [75]. Superhydrophobic coatings have shown excellent corrosion resistance in highly acidic or aggressive media [76]. The fabricated hierarchical structures offer a passivation layer hindering metallic corrosion, e.g., offshore wind turbines subjected to aggressive environments with high humidity and intensive salinity, offering a new efficient mechanism from anti-corrosion. Liu et al. [77] stated that the anti-corrosion mechanism of superhydrophobic coatings under salty water is avoiding corrosive medium like Cl− reaching the metal substrate.
While superhydrophobic coatings have excellent anti-corrosion features, chemical corrosion could also lead to the degradation of hydrophobicity. An important chemical corrosion (or called contamination) subjected to aircraft ground icing is the corrosion of deicing fluids to superhydrophobic coatings. Aircraft ground deicing often involves using chemicals such as deicers or anti-icing agents. While these chemicals aid in ice removal, they can also degrade the performance of superhydrophobic coating by resolving the coating material or depositing hydrophilic agents on the surface since deicing fluids usually contain glycols and other organic solvents [42]. Zhang et al. [42] found that a commercial type I deicing fluid mainly containing propylene glycol could degrade a silicon-based superhydrophobicity by resolving the topping material and significantly undermining the hierarchical structure. Furthermore, the thickening agents (e.g., xanthan gum) in a type IV deicing fluid could adhere tightly on superhydrophobic coatings, which results in the transition of surface wettability from superhydrophobic to hydrophilic very quickly [42]. Even though the coating intrinsic chemical characteristics are not altered in this case, the surface is covered by another hydrophilic material in the aircraft deicing process, which should be cautious for the application of superhydrophobic coatings on aircraft.
4.3 UV irradiation
In the presence of oxygen or water in the environment, UV in sunlight could induce the photo-oxidation of coatings. The UV resistance of superhydrophobic coatings varies with the coating composition. Shorter wavelengths in the UV range result in more severe damage to the opaque coating, whereas UV-transparent coatings can very well resist the photo-oxidation induced by UV light. Superhydrophobic coatings that only absorb UV light with wavelengths lower than 280 nm usually have good durability to resist UV radiation [33].
The UV durability of superhydrophobic coatings could be assessed by placing the coatings under sunlight in order to cover the whole range of sunlight spectrum but this method is not time efficient [78]. A climate chamber equipped with an intensive UV light lamp can accelerate the degradation of superhydrophobic coating [79]. An international standard, that is, ASTM G154, has regulated the standard practice for operating UV lamp for exposure of non-metallic materials, which is widely used in many previous studies of testing UV degradation of superhydrophobic coatings [80, 81].
Plenty of studies are dedicated to fabricating UV-resistant superhydrophobic coatings. Radwan et al. [82] combined poly(vinylidene fluoride-co-hexafluoropropylene) with alumina nanoparticles to create a superhydrophobic coating with high UV durability. Their results showed that after a long 500-hour UV exposure at a constant temperature of 60
4.4 Insect contamination
Insect impact are operational events of aircraft occurring during the ground operation, take-off and landing phases of the flight profile, which was recognized as early as 1945. Insect impact residue can greatly contaminate the aircraft surface (see Figure 9(a)) and alter the aerodynamic performance of aircraft [86]. The issue of insect debris has garnered attention in multiple industries. Specifically, it has been a focus for the wind farm industry. There has been a particular interest in understanding the effect of surface irregularities induced by the insect contamination (see Figure 9(b)) on the efficiency of wind turbine blades and the subsequent power losses they may cause. Corten and Veldkamp [85] hypothesized that the insect debris at the suction peak of turbine blades could reduce the power output and verified this hypothesis experimentally (see Figure 9(c)).
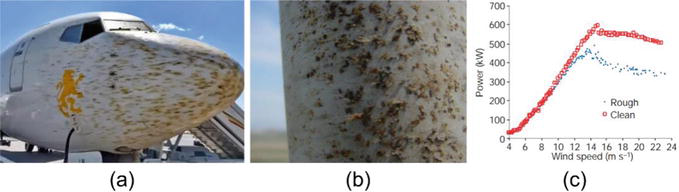
Figure 9.
Insect contamination on an (a) aircraft and a (b) wind turbine. (c) Insect-contaminated wind turbine generates less power than the clean turbine [
Superhydrophobic coatings are effective in reducing insect contamination by significantly decreasing the adhesion of the debris. This property makes it difficult for insects to adhere to the coating. Krishnan et al. [87] observed that the superhydrophobic coating could reduce the contamination area efficiently since the superhydrophobic coating significantly promotes the retracting of insect debris. However, they also pointed out that superhydrophobic and oleophobic coatings are ideal for minimizing insect contamination because the adhesion of insect blood is low on oleophobic coatings rather than superhydrophobic coatings. Kok et al. [88] tested the ability of contamination cleaning for surfaces with various roughness and proposed that rougher surfaces with better hydrophobicity display less residue adhesion.
5. Recommendations for the durability of superhydrophobic coatings pertinent to aircraft/wind turbine icing mitigation
This chapter reviews the recent advances in durability of superhydrophobic coatings pertinent to aircraft and wind turbine icing mitigation. In order to develop effective superhydrophobic coating for aircraft/wind turbines, it is necessary to have a consistent, reliable method for characterizing the performance of candidate materials subjected to realistic environmental conditions, such as rain, sand and ultraviolet exposure. As a result, the durability tests listed above (i.e., droplet erosion resistance, sand erosion resistance, abrasion resistance, icing/deicing resistance, UV resistance, chemical corrosion resistance and insect contamination) are necessary to be conducted to quantify the durability of superhydrophobic coatings before applying them on aircraft and wind turbines in practice. This chapter is intended to display the problem and perhaps can shed some light into conducting tests that can be industrially valuable. Here are some recommendations for the durability test of superhydrophobic coatings:
In order to assist the advances in the application of superhydrophobic coatings, it is significant to standardize the evaluation methods based on the working conditions of aircraft and wind turbines. This chapter indicates that some of the above durability testing methods are well studied in this regard, and this allows specific recommendations. Table 1 summarizes the standard methods for durability assessment of a coating. However, there is currently a lack of specific durability testing standards for superhydrophobic coatings that can be applied to aircraft or wind turbines. As a result, some standards or regulations for coating durability assessment should be developed or updated based on current standards. For example, ASTM G73 regulates the droplet erosion durability of coatings by quantifying the material removal rate. However, superhydrophobic coatings suffer degradation (e.g., a decrease of the static contact angle) after the droplet erosion test with little material removal. In this way, ASTM G73 should be updated to include the hydrophobicity degradation after the droplet erosion test to meet the specific requirement of applications of superhydrophobic coatings on aircraft.
The importance of each durability test needs to be quantified based on local environmental conditions since natural factors that influence the durability of superhydrophobic coating vary with different regions. For example, sand erosion is severe in Middle Eastern countries where the desert covers a large portion of countries, but it is not a primary concern in countries in Western European countries that receive a significant amount of rainfall throughout the year from the Atlantic Ocean. Instead, superhydrophobic coatings prepared for offshore wind turbines in Europe are severely threatened by the corrosion of salty vapor and droplet erosion due to plenty of airborne tiny droplets.
The durability tests of superhydrophobic coatings should be application specific. Fabricating a superhydrophobic, durable coating for all tests listed above is an overwhelming task. Despite this fact, the coating should also be excellent if it is durable under the situations where it mostly works. Offshore wind turbines mostly work in aggressive environments with high humidity, severe corrosion and intense UV radiation but sand erosion is rare. As a result, developing a superhydrophobic coating with excellent UV and corrosion resistance is an ideal coating for the icing mitigation of offshore wind turbines. Instead, aircraft working in airports in the desert faces intense UV radiation in park and severe sand erosion during landing and take-off, so a superhydrophobic coating with excellent erosion and UV resistance would be suitable for aircraft icing mitigation.
Coating durability needs to be further improved to allow the coating to perform well in operating conditions of aircraft and wind turbines. The coating should be tested in a realistic and dynamic environment, such as inside a droplet erosion test rig where the droplet can impinge onto the coating with a speed over 100 m/s analogous to the landing or take-off condition.
Durability type | Standard code | Test details |
---|---|---|
Droplet erosion | ASTM G73 | Samples are installed on a rotating arm and impinge with droplets at high speed (up to 600 m/s). |
Sand erosion | ASTM G76 | Samples are eroded by high-speed airborne particles injected by a nozzle with a specified particle size, velocity, and attack angle. |
Mechanical abrasion | ASTM D4060 | Abrasion of samples with different loads and abraders. |
Chemical corrosion | ASTM G1654 | Evaluating basic corrosion performance of coatings after exposure to corrosive environments. |
UV | ASTM G154 | Simulation of weathering conditions in a chamber under consecutive UV irradiation. |
Icing/deicing cycles | ASTM C1645 | Determining the effects of icing/deicing cycles on the length of service of concrete paving units. |
Table 1.
6. Conclusions
In conclusion, superhydrophobic coatings that can create large microcracks have demonstrated significant potential in anti-icing applications. These coatings exhibit excellent water repellency, creating a protective barrier on surfaces that prevent ice formation. Superhydrophobic coatings can effectively reduce ice accumulation and enhance anti-icing properties by minimizing the adhesion of water droplets/ice. Compared to conventional thermal-based anti-icing technologies, superhydrophobic coatings have advantages of lightweight and low energy consumption. As a result, applying superhydrophobic coatings to achieve surface modification is a promising anti-icing technique in wide applications including aerospace and wind energy. International Organization for Standardization (ISO) has published a standard (ISO10689) to systematically evaluate the durability of superhydrophobic coatings pertinent to industrial applications.
While superhydrophobic coatings show promising results in anti-icing applications, further research is still needed to optimize their performance and extend the length of service. This chapter mainly reviews the challenges in improving the durability of superhydrophobic coatings and highlights recent advances in enhancing the coating durability. One of the challenges is droplet erosion, which could occur on in-flight aircrafts or offshore wind turbines subject to the high-speed impact of droplets. The “water hammer effect” in the high-speed impact generates tremendous stress, leading to severe coating damage. Further, our recent study indicates that the water hammer pressure and Rayleigh surface wave induced by the impact are the main factors leading to the coating degradation in droplet erosion. Sand erosion, mechanical abrasion and icing/deicing test also significantly change the surface topology, but UV radiation and chemical corrosion change the surface chemistry. In this way, the durability of superhydrophobic coatings with hierarchical structures can be defined as the ability of a surface to maintain surface topology and low surface energy under practical working conditions. As a result, durable superhydrophobic coatings should be fabricated with excellent mechanical hardness and stable chemical properties.
Acknowledgments
The author would like to thank the support of Zhongfa Aviation Institute of Beihang University.
References
- 1.
Cao Y, Tan W, Wu Z. Aircraft icing: An ongoing threat to aviation safety. Aerospace Science and Technology. 2018; 75 :353-385. DOI: 10.1016/j.ast.2017.12.028 - 2.
De Pauw D, Dolatabadi A. Effect of superhydrophobic coating on the anti-icing and deicing of an airfoil. Journal of Aircraft. 2017; 54 :490-499. DOI: 10.2514/1.C033828 - 3.
Han N, Siddique MA, Zhang Z, Tian L, Hu H, Hu H. A flight-testing campaign to examine inflight icing characteristics and its effects on the flight performance of an unmanned-aerial-vehicle. Cold Regions Science and Technology. 2023; 207 :103775. DOI: 10.1016/j.coldregions.2023.103775 - 4.
Botura G, Fahrner A. Icing Detection System–Conception, Development, Testing and Applicability to UAVs. San Diego, California: Goodrich Corp AIAA; 2003 - 5.
Zhang B, Tang L, Roemer M. Probabilistic weather forecasting analysis for unmanned aerial vehicle path planning. Journal of Guidance, Control, and Dynamics. 2014; 37 :309-312. DOI: 10.2514/1.61651 - 6.
Li L, Liu Y, Tian L, Hu H, Hu H, Liu X, et al. An experimental study on a hot-air-based anti- /de-icing system for aero-engine inlet guide vanes. Applied Thermal Engineering. 2020; 167 :114778. DOI: 10.1016/j.applthermaleng.2019.114778 - 7.
Liu Y, Kolbakir C, Hu H, Meng X, Hu H. An experimental study on the thermal effects of duty-cycled plasma actuation pertinent to aircraft icing mitigation. International Journal of Heat and Mass Transfer. 2019; 136 :864-876. DOI: 10.1016/j.ijheatmasstransfer.2019.03.068 - 8.
Muthumani A, Fay L, Akin M, Wang S, Gong J, Shi X. Correlating lab and field tests for evaluation of deicing and anti-icing chemicals: A review of potential approaches. Cold Regions Science and Technology. 2014; 97 :21-32. DOI: 10.1016/j.coldregions.2013.10.001 - 9.
Gao L, Liu Y, Ma L, Hu H. A hybrid strategy combining minimized leading-edge electric-heating and superhydro-/ice-phobic surface coating for wind turbine icing mitigation. Renewable Energy. 2019; 140 :943-956. DOI: 10.1016/j.renene.2019.03.112 - 10.
Huang X, Tepylo N, Pommier-Budinger V, Budinger M, Bonaccurso E, Villedieu P, et al. A survey of icephobic coatings and their potential use in a hybrid coating/active ice protection system for aerospace applications. Progress in Aerospace Science. 2019; 105 :74-97. DOI: 10.1016/j.paerosci.2019.01.002 - 11.
Cohen N, Dotan A, Dodiuk H, Kenig S. Superhydrophobic coatings and their durability. Materials and Manufacturing Processes. 2016; 31 :1143-1155. DOI: 10.1080/10426914.2015.1090600 - 12.
Hooda A, Goyat MS, Pandey JK, Kumar A, Gupta R. A review on fundamentals, constraints and fabrication techniques of superhydrophobic coatings. Progress in Organic Coatings. 2020; 142 :105557. DOI: 10.1016/j.porgcoat.2020.105557 - 13.
Yang N, Li J, Bai N, Xu L, Li Q. One step phase separation process to fabricate superhydrophobic PVC films and its corrosion prevention for AZ91D magnesium alloy. Materials Science and Engineering B: Solid-State Materials for Advanced Technology. 2016; 209 :1-9. DOI: 10.1016/j.mseb.2016.01.009 - 14.
Ding B, Ogawa T, Kim J, Fujimoto K, Shiratori S. Fabrication of a super-hydrophobic nanofibrous zinc oxide film surface by electrospinning. Thin Solid Films. 2008; 516 :2495-2501. DOI: 10.1016/j.tsf.2007.04.086 - 15.
Yang Y, He H, Li Y, Qiu J. Using nanoimprint lithography to create robust, buoyant, superhydrophobic PVB/SiO2 coatings on wood surfaces inspired by red roses petal. Scientific Reports. 2019; 9 :1-9. DOI: 10.1038/s41598-019-46337-y - 16.
Zhu X, Zhang Z, Ren G, Yang J, Wang K, Xu X, et al. A novel superhydrophobic bulk material. Journal of Materials Chemistry. 2012; 22 :20146-20148. DOI: 10.1039/c2jm33769j - 17.
Singh AK, Singh JK. Fabrication of durable superhydrophobic coatings on cotton fabrics with photocatalytic activity by fluorine-free chemical modification for dual-functional water purification. New Journal of Chemistry. 2017; 41 :4618-4628. DOI: 10.1039/c7nj01042g - 18.
Wang D, Sun Q , Hokkanen MJ, Zhang C, Lin FY, Liu Q , et al. Design of robust superhydrophobic surfaces. Nature. 2020; 582 :55-59. DOI: 10.1038/s41586-020-2331-8 - 19.
Li L, Roethel S, Breedveld V, Hess DW. Creation of low hysteresis superhydrophobic paper by deposition of hydrophilic diamond-like carbon films. Cellulose. 2013; 20 :3219-3226. DOI: 10.1007/s10570-013-0078-1 - 20.
Leary WM. We Freeze to Please: A History of NASA’s Icing Research Tunnel and the Quest for Flight Safety. Vol. 4226. National Aeronautics and Space Administration; 2002 - 21.
Struk P, Broeren A, Potapczuk M, Kreeger RE, Blankenship K, Chen R-C, et al. NASA Analysis of Alternatives Study for Icing Research. Cleveland, Ohio, USA: National Aeronautics and Space Administration; 2022 - 22.
Sojoudi H, Wang M, Boscher ND, McKinley GH, Gleason KK. Durable and scalable icephobic surfaces: Similarities and distinctions from superhydrophobic surfaces. Soft Matter. 2016; 12 :1938-1963. DOI: 10.1039/c5sm02295a - 23.
Milionis A, Loth E, Bayer IS. Recent advances in the mechanical durability of superhydrophobic materials. Advances in Colloid and Interface Science. 2016; 229 :57-79. DOI: 10.1016/j.cis.2015.12.007 - 24.
Gorb SN, Gorb EV. Anti-icing strategies of plant surfaces: The ice formation on leaves visualized by cryo-SEM experiments. Science of Nature. 2022; 109 :24. DOI: 10.1007/s00114-022-01789-7 - 25.
Byun S, Jeong H, Kim DR, Lee KS. Frost layer growth behavior on ultra-low temperature surface with a superhydrophobic coating. International Communications in Heat and Mass Transfer. 2021; 128 :105641. DOI: 10.1016/j.icheatmasstransfer.2021.105641 - 26.
Zhang Z, Ma L, Liu Y, Ren J, Hu H. An experimental study of rain erosion effects on a hydro-/ice-phobic coating pertinent to unmanned-arial-system (UAS) inflight icing mitigation. Cold Regions Science and Technology. 2021; 181 :103196. DOI: 10.1016/j.coldregions.2020.103196 - 27.
Gohardani O. Impact of erosion testing aspects on current and future flight conditions. Progress in Aerospace Science. 2011; 47 :280-303. DOI: 10.1016/j.paerosci.2011.04.001 - 28.
Tian X, Verho T, Ras RHA. Moving superhydrophobic surfaces toward real-world applications. Science (80- ). 2016; 352 :142-143. DOI: 10.1126/science.aaf2073 - 29.
Wang L, Tian Z, Jiang G, Luo X, Chen C, Hu X, et al. Spontaneous dewetting transitions of droplets during icing & melting cycle. Nature Communications. 2022; 13 :378. DOI: 10.1038/s41467-022-28036-x - 30.
Ma L, Zhang Z, Gao L, Liu Y, Hu H. An exploratory study on using slippery-liquid-infused-porous-surface (SLIPS) for wind turbine icing mitigation. Renewable Energy. 2020; 162 :2344-2360. DOI: 10.1016/j.renene.2020.10.013 - 31.
Xiu Y, Liu Y, Hess DW, Wong CP. Mechanically robust superhydrophobicity on hierarchically structured Si surfaces. Nanotechnology. 2010; 21 :155705. DOI: 10.1088/0957-4484/21/15/155705 - 32.
Myronyuk O, Baklan D, Yong Z, Rodin AM. Complex destruction of textured water-repellent coatings under the influence of UV and water flow. Materials Today Communications. 2022; 33 :104509. DOI: 10.1016/j.mtcomm.2022.104509 - 33.
Hakimian A, Nazifi S, Ghasemi H. Durability assessment of icephobic coatings. In: Mittal KL, Choi CH, editors. Ice Adhesion. 2020. DOI: 10.1002/9781119640523.ch17 - 34.
Dalawai SP, Saad Aly MA, Latthe SS, Xing R, Sutar RS, Nagappan S, et al. Recent advances in durability of superhydrophobic self-cleaning technology: A critical review. Progress in Organic Coatings. 2020; 138 :105381. DOI: 10.1016/j.porgcoat.2019.105381 - 35.
Chen H, Muros-Cobos JL, Amirfazli A. Contact angle measurement with a smartphone. The Review of Scientific Instruments. 2018; 89 (3):035117. DOI: 10.1063/1.5022370 - 36.
Huang X, Gates I. Apparent contact angle around the periphery of a liquid drop on roughened surfaces. Scientific Reports. 2020; 10 :8220. DOI: 10.1038/s41598-020-65122-w - 37.
Schrader ME. Young-dupre revisited. Langmuir. 1995; 11 :3585-3589. DOI: 10.1021/la00009a049 - 38.
Yang C, Zeng Q , Huang J, Guo Z. Droplet manipulation on superhydrophobic surfaces based on external stimulation: A review. Advances in Colloid and Interface Science. 2022; 306 :102724. DOI: 10.1016/j.cis.2022.102724 - 39.
Work A, Lian Y. A critical review of the measurement of ice adhesion to solid substrates. Progress in Aerospace Science. 2018; 98 :1-26. DOI: 10.1016/j.paerosci.2018.03.001 - 40.
Meuler AJ, Smith JD, Varanasi KK, Mabry JM, McKinley GH, Cohen RE. Relationships between water wettability and ice adhesion. ACS Applied Materials & Interfaces. 2010; 2 :3100-3110. DOI: 10.1021/am1006035 - 41.
Butt HJ, Liu J, Koynov K, Straub B, Hinduja C, Roismann I, et al. Contact angle hysteresis. Current Opinion in Colloid & Interface Science. 2022; 59 :101574. DOI: 10.1016/j.cocis.2022.101574 - 42.
Zhang Z, Lusi A, Hu H, Bai X, Hu H. An experimental study on the detrimental effects of deicing fluids on the performance of icephobic coatings for aircraft icing mitigation. Aerospace Science and Technology. 2021; 119 :107090. DOI: 10.1016/j.ast.2021.107090 - 43.
Zhang Z, Ou J, Li W, Amirfazli A. Folding characteristics of membranes in capillary origami. Journal of Colloid and Interface Science. 2023; 630 :111-120. DOI: 10.1016/j.jcis.2022.10.046 - 44.
Bengaluru Subramanyam S, Kondrashov V, Rühe J, Varanasi KK. Low ice adhesion on nano-textured superhydrophobic surfaces under supersaturated conditions. ACS Applied Materials & Interfaces. 2016; 8 :12583-12587. DOI: 10.1021/acsami.6b01133 - 45.
Herring R, Dyer K, Martin F, Ward C. The increasing importance of leading edge erosion and a review of existing protection solutions. Renewable and Sustainable Energy Reviews. 2019; 115 :109382. DOI: 10.1016/j.rser.2019.109382 - 46.
Keegan MH, Nash DH, Stack MM. On erosion issues associated with the leading edge of wind turbine blades. Journal of Physics D: Applied Physics. 2013; 46 :383001. DOI: 10.1088/0022-3727/46/38/383001 - 47.
Zhan Y, Yu S, Amirfazli A, Rahim Siddiqui A, Li W. Recent advances in antibacterial superhydrophobic coatings. Advanced Engineering Materials. 2022; 24 :1-22. DOI: 10.1002/adem.202101053 - 48.
Fujisawa N, Takano S, Fujisawa K, Yamagata T. Experiments on liquid droplet impingement erosion on a rough surface. Wear. 2018; 398-399 :158-164. DOI: 10.1016/j.wear.2017.12.003 - 49.
Ghidaoui MS, Zhao M, McInnis DA, Axworthy DH. A review of water hammer theory and practice. Applied Mechanics Reviews. 2005; 58 :49. DOI: 10.1115/1.1828050 - 50.
Field JE. The physics of liquid impact, shock wave interactions with cavities, and the implications to shock wave lithotripsy. Physics in Medicine and Biology. 1991; 36 :1475-1484. DOI: 10.1088/0031-9155/36/11/007 - 51.
Lesser MB. Analytic solutions of liquid-drop impact problems. Proceedings of the Royal Society A: Mathematical, Physical and Engineering Sciences. 1981; 377 :289-308. DOI: 10.1098/rspa.1981.0125 - 52.
Soto D, De Larivière AB, Boutillon X, Clanet C, Quéré D. The force of impacting rain. Soft Matter. 2014; 10 :4929-4934. DOI: 10.1039/C4SM00513A - 53.
Grundwürmer M, Nuyken O, Meyer M, Wehr J, Schupp N. Sol-gel derived erosion protection coatings against damage caused by liquid impact. Wear. 2007; 263 :318-329. DOI: 10.1016/j.wear.2006.12.039 - 54.
Blowers RM. On the response of an elastic solid to droplet impact. IMA Journal of Applied Mathematics. 1969; 5 :167-193. DOI: 10.1093/imamat/5.2.167 - 55.
Athanasopoulos GA, Pelekis PC, Anagnostopoulos GA. Effect of soil stiffness in the attenuation of Rayleigh-wave motions from field measurements. Soil Dynamics and Earthquake Engineering. 2000; 19 :277-288. DOI: 10.1016/S0267-7261(00)00009-9 - 56.
Brown S, Lengaigne J, Sharifi N, Pugh M, Moreau C, Dolatabadi A, et al. Durability of superhydrophobic duplex coating systems for aerospace applications. Surface and Coatings Technology. 2020; 401 :126249. DOI: 10.1016/j.surfcoat.2020.126249 - 57.
Song N, Benmeddour A. Erosion resistant hydrophobic coatings for passive ice protection of aircraft. Applied Sciences. 2022; 12 :9589. DOI: 10.3390/app12199589 - 58.
Chen J, Wang J, Ni A. A review on rain erosion protection of wind turbine blades. Journal of Coatings Technology and Research. 2019; 16 :15-24. DOI: 10.1007/s11998-018-0134-8 - 59.
Meena MK, Tudu BK, Kumar A, Bhushan B. Development of polyurethane-based superhydrophobic coatings on steel surfaces. Philosophical Transactions of the Royal Society A—Mathematical Physical and Engineering Sciences. 2020; 378 :20190446. DOI: 10.1098/rsta.2019.0446 - 60.
Hutchings IM, Winter RE. Particle erosion of ductile metals: A mechanism of material removal. Wear. 1974; 27 :121-128. DOI: 10.1016/0043-1648(74)90091-X - 61.
Wang YF, Yang ZG. Finite element model of erosive wear on ductile and brittle materials. Wear. 2008; 265 :871-878. DOI: 10.1016/j.wear.2008.01.014 - 62.
Wood RJK. The sand erosion performance of coatings. Materials and Design. 1999; 20 :179-191. DOI: 10.1016/s0261-3069(99)00024-2 - 63.
Kumar D, Li L, Chen Z. Mechanically robust polyvinylidene fluoride (PVDF) based superhydrophobic coatings for self-cleaning applications. Progress in Organic Coatings. 2016; 101 :385-390. DOI: 10.1016/j.porgcoat.2016.09.003 - 64.
Fu J, Sun Y, Ji Y, Zhang J. Fabrication of robust ceramic based superhydrophobic coating on aluminum substrate via plasma electrolytic oxidation and chemical vapor deposition methods. Journal of Materials Processing Technology. 2022; 306 :117641. DOI: 10.1016/j.jmatprotec.2022.117641 - 65.
Zheng S, Bellido-Aguilar DA, Huang Y, Zeng X, Zhang Q , Chen Z. Mechanically robust hydrophobic bio-based epoxy coatings for anti-corrosion application. Surface and Coatings Technology. 2019; 363 :43-50. DOI: 10.1016/j.surfcoat.2019.02.020 - 66.
Hensel R, Finn A, Helbig R, Braun HG, Neinhuis C, Fischer WJ, et al. Biologically inspired omniphobic surfaces by reverse imprint lithography. Advanced Materials. 2014; 26 :2029-2033. DOI: 10.1002/adma.201305408 - 67.
Kondrashov V, Rühe J. Microcones and nanograss: Toward mechanically robust superhydrophobic surfaces. Langmuir. 2014; 30 :4342-4350. DOI: 10.1021/la500395e - 68.
Dong Z, Vuckovac M, Cui W, Zhou Q , Ras RHA, Levkin PA. 3D printing of superhydrophobic objects with bulk nanostructure. Advanced Materials. 2021; 33 :2106068. DOI: 10.1002/adma.202106068 - 69.
Huang J, Li M, Ren C, Huang W, Miao Y, Wu Q , et al. Construction of HLNPs/Fe3O4based superhydrophobic coating with excellent abrasion resistance, UV resistance, flame retardation and oil absorbency. Journal of Environmental Chemical Engineering. 2023; 11 :109046. DOI: 10.1016/j.jece.2022.109046 - 70.
Dong S, Yang Y, Ma R, Du A, Yang M, Fan Y, et al. Investigation of the anti-corrosion performance of a Cu/Ni–B4C superhydrophobic coating with self-cleaning and abrasion resistance properties. Surface and Coatings Technology. 2022; 441 :128568. DOI: 10.1016/j.surfcoat.2022.128568 - 71.
Hu H, Tian L, Hu H. Experimental investigation on ice accretion upon ice particle impacting onto heated surface. AIAA Journal. 2023; 61 :3019-3031. DOI: 10.2514/1.j062425 - 72.
Foroughi Mobarakeh L, Jafari R, Farzaneh M. The ice repellency of plasma polymerized hexamethyldisiloxane coating. Applied Surface Science. 2013; 284 :459-463. DOI: 10.1016/j.apsusc.2013.07.119 - 73.
Wang Y, Xue J, Wang Q , Chen Q , Ding J. Verification of icephobic/anti-icing properties of a superhydrophobic surface. ACS Applied Materials & Interfaces. 2013; 5 :3370-3381. DOI: 10.1021/am400429q - 74.
Tetteh E, Loth E. Reducing static and impact ice adhesion with a self-lubricating icephobic coating (SLIC). Coatings. 2020; 10 :262. DOI: 10.3390/coatings10030262 - 75.
Wang ZM, Song GL, Zhang J. Corrosion control in CO2 enhanced oil recovery from a perspective of multiphase fluids. Frontiers in Materials. 2019; 6 :1-16. DOI: 10.3389/fmats.2019.00272 - 76.
Gupta R, Verma R, Kango S, Constantin A, Kharia P, Saini R, et al. A critical review on recent progress, open challenges, and applications of corrosion-resistant superhydrophobic coating. Materials Today Communications. 2023; 34 :105201. DOI: 10.1016/j.mtcomm.2022.105201 - 77.
Liu T, Yin Y, Dong L. New application of the “underwater super-hydrophobic” surface in the corrosion protection. Advances in Materials Research. 2009; 79-82 :1115-1118. DOI: 10.4028/www.scientific.net/AMR.79-82.1115 - 78.
Irajizad P, Nazifi S, Ghasemi H. Icephobic surfaces: Definition and figures of merit. Advances in Colloid and Interface Science. 2019; 269 :203-218. DOI: 10.1016/j.cis.2019.04.005 - 79.
Sataeva NE, Boinovich LB, Emelyanenko KA, Domantovsky AG, Emelyanenko AM. Laser-assisted processing of aluminum alloy for the fabrication of superhydrophobic coatings withstanding multiple degradation factors. Surface and Coatings Technology. 2020; 397 :125993. DOI: 10.1016/j.surfcoat.2020.125993 - 80.
Allahdini A, Momen G, Munger F, Brettschneider S, Fofana I, Jafari R. Performance of a nanotextured superhydrophobic coating developed for high-voltage outdoor porcelain insulators. Colloids and Surfaces A: Physicochemical and Engineering Aspects. 2022; 649 :129461. DOI: 10.1016/j.colsurfa.2022.129461 - 81.
Foroughi Mobarakeh L, Jafari R, Farzaneh M. Robust icephobic, and anticorrosive plasma polymer coating. Cold Regions Science and Technology. 2018; 151 :89-93. DOI: 10.1016/j.coldregions.2018.03.009 - 82.
Radwan AB, El-Hout SI, Ibrahim MAM, Ismail EH, Abdullah AM. Superior corrosion and UV-resistant highly porous poly(vinylidene fluoride- co-hexafluoropropylene)/alumina superhydrophobic coating. ACS Applied Polymer Materials. 2022; 4 :1358-1367. DOI: 10.1021/acsapm.1c01710 - 83.
Raghu SNV, Chuluunbandi K, Killian MS. Zirconia nanotube coatings—UV-resistant superhydrophobic surfaces. Surfaces and Interfaces. 2021; 26 :101357. DOI: 10.1016/j.surfin.2021.101357 - 84.
Gao Y, Gereige I, El Labban A, Cha D, Isimjan TT, Beaujuge PM. Highly transparent and UV-resistant superhydrophobic SiO2-coated ZnO nanorod arrays. ACS Applied Materials & Interfaces. 2014; 6 :2219-2223. DOI: 10.1021/am405513k - 85.
Corten GP, Veldkamp HF. Aerodynamics: Insects can halve wind-turbine power. Nature. 2001; 412 :41-42. DOI: 10.1038/35083698 - 86.
Kok M, Smith JG, Wohl CJ, Siochi EJ, Young TM. Critical considerations in the mitigation of insect residue contamination on aircraft surfaces—A review. Progress in Aerospace Science. 2015; 75 :1-14. DOI: 10.1016/j.paerosci.2015.02.001 - 87.
Krishnan KG, Milionis A, Loth E, Farrell TE, Crouch JD, Berry DH. Influence of hydrophobic and superhydrophobic surfaces on reducing aerodynamic insect residues. Applied Surface Science. 2017; 392 :723-731. DOI: 10.1016/j.apsusc.2016.09.096 - 88.
Kok M, Mertens T, Raps D, Young TM. Influence of surface characteristics on insect residue adhesion to aircraft leading edge surfaces. Progress in Organic Coatings. 2013; 76 :1567-1575. DOI: 10.1016/j.porgcoat.2013.06.013