Search terms and limitations used in article searches.
Abstract
Mesenchymal stromal cells (MSCs) are known for their immunomodulatory properties, and their role in antiviral response is poorly understood. The susceptibility of the MSCs to viral infection or viral tropism toward MSCs can be emanated from few available literature evidences. What makes MSCs special is the ability to sustain infection and reciprocate through immune intermediates like antimicrobial peptides, cytokines, and secretomes. However, care has to be taken to understand that MSCs can transmit viral infections and are known for their vulnerability to many microorganisms in general. In the recent past, after deadly infections like Ebola, Zika, and HIV, COVID-19 had posed a great threat, where stem cell transplantation was a suggestive therapeutic model in some cases due to the cytokine storm and other additional biochemical, molecular, and transcriptional factors associated with the pathology. This is true in many other common viral infections at large. In this chapter, the role of MSCs in combating viral infections as well as their susceptibility pattern are discussed. Further, the role of MSCs in immunomodulation and their antiviral factors cannot be delineated in understanding the immunological mechanisms preventing tissue damages associated with viral infection.
Keywords
- MSCs
- immunomodulation
- mesenchymal stromal cells
- secretomes
- antiviral response
1. Introduction
Among the numerous forms of infectious diseases worldwide, viral infections have been the biggest known threat in the recent years [1]. The spread of a new coronavirus (COVID-19) caused by severe acute respiratory syndrome coronavirus (SARS-CoV-2) affected more than 110 million people worldwide. It has prompted the scientific world to prevent and treat by developing of new vaccination or through antiviral drugs [2]. Mesenchymal stromal cells (MSCs) constitute a heterogeneous population of immunoregulatory stem cells that are known to be highly regenerative and antiviral immune response [3]. They replicate vigorously in culture plates and maintain their biological properties. MSCs were isolated from many tissues around the body such as the placenta, adipose tissues and bone marrow. Besides their nature, MSCs have wide clinical usage that includes inflammatory diseases, myocardial infarction, degenerative disorders, and pneumonia [4]. Nevertheless, MSCs have good antiviral properties and are involved in the treatment of viral infection in the last few years. Immediately after viral invasion, damaged associated molecular patterns (DAMPs) and/or pathogen-associated molecular patterns (PAMPs) induce pro-inflammatory (MSC1) phenotypes in MSCs [5] and regulate circulatory immune cells involved in antiviral response. The International Society of Cell Therapy (ISCT) has established universal criteria for MSC definition. Therefore, MSCs must display plastic-adherence capacity; fibroblastic spindle shape morphology in standard culture media; surface expression of CD90, CD73, and CD105 and absence of CD11b, CD34, CD45, and HLA-DR; and
MSCs are known to interact with various types of immune cells such as dendritic cells (DCs), macrophages, Natural Killer cells (NK) B-lymphocytes, CD4+, T helper cells, and cytotoxic T lymphocytes (CTLs) [5]. MSCs are known to inhibit NK cell and T-cell proliferation and reduce the differentiation of B cells to antibody-secreting plasma cells [7]. MSC-sourced interferons (IFNs) modulate the cytotoxic properties of NK cells, and CTLs enhance antigen-presenting properties of DCs. Macrophages and B cells contribute to the effective removal of virus-infected cells [8]. However, the activities of MSCs change due to influence of the local microenvironment, which leads to complexity in understanding the MSC-mediated immune response. Due to their potential immunomodulation and systemic inflammatory responses, MSCs are in a large number of clinical and experimental studies, exploring a wide area of new approach in the treatment of viral diseases [9]. In this book chapter, we summarized the current knowledge on MSC-dependent cellular mechanisms that are involved in the elimination of viruses, modulation of immune responses, and repair and regeneration of tissue damages caused by viral pathogens, and the efficacy of clinical practices about MSC-mediated therapy was put forth and evaluated.
2. Susceptibility of viral infection to MSCs
MSCs are prone to DNA (Deoxyribonucleic acid) or RNA (Ribonucleic acid) viruses both
The parvovirus B19 is a DNA virus (single-stranded) that infects bone marrow BM-MSCs of human with upregulation of pro-inflammatory cytokine gene expression, such as IL-6 and TNF-α [19]. Cytokine-induced immunomodulatory function was lost when MSCs were infected with CMV and no longer inhibited microbial growth [20]. Also, the US11 protein used by CMV for immune invasion downregulated MHC class I expression of human MSCs, making them vulnerable to NK cell-mediated lysis [21]. A similar effect was obtained when horse MSCs was infected with equid herpesvirus-1 (EHV-1). The ability of viruses that invade and infect a cell varies between host types and is species-dependent. For instance, different MSCs of two closely related species human and murine produce distinct varied immunomodulatory mediators, and this indeed regulates the chance to limit or enhance the viral replication is an important concern. Indoleamine-2,3-dioxygenase (IDO) is known to be a primary mediator for viral replication in human MSCs, and it was found to have no effect in murine MSCs [22].
3. Antiviral response of MSCs
Despite the virus permissiveness, evidence has emerged that MSCs can mitigate viral infection
4. Effect of MSCs on cell-based immune response
Besides their intrinsic inhibition to viruses, MSCs could modify the antiviral response of immune cells as they normally do with antiviral defenses. However, these interactions influence both innate and adaptive immune components
5. Engineered MSC-EVs as therapeutic vehicles
Mesenchymal stem cell-derived extracellular vesicles (MSC-EVs) represent potential cell-free alternative to stem cell therapy but are also rapidly emerging as a novel therapeutic platform particularly in the form of engineered EVs (EEVs) tailored to target a broad range of clinical indications. Several biodistribution studies using labeled dyes for EV uptake into animal models proved the accumulation of 70% dyes in the liver and spleen [33, 34]. However, the engineered EVs facilitate the distribution of therapeutic molecules into other organs and were considered by some biopharma companies “hard to treat disease,” for example, cancer [35]. One method conferring this is to alter selected cell-surface-proteins. One route is to produce a fusion protein that inserts its tail into the EV membrane and the head binds to a projecting receptor (Figure 1) [37]. Modified EEVs could be accomplished by favoring selected cell surface protein alteration that confers target capabilities. One such copy was used to target neuron cells in the brain after systemic injection. The fusion protein was created between EV membrane protein Lam2b and rabies viral glycoprotein (RVG) peptide, which binds the acetylcholine receptor on the brain cells [36]. Tian et al. [38] have shown that cultured proteins from cell cultures can also be incorporated onto EVs for target delivery. A recent study has showed that fusion proteins that bind the phosphatidylserine onto EVs to ischemic brain tissues after systemic injection relieving inflammation. Similar approaches were also used to treat tumor cells in a mouse model of glioblastoma [39]. EEVs with protein receptors on their surface have been developed as decoys to capture target molecules such as the pro-inflammatory cytokine IL-6 as a potential therapeutic for chronic inflammatory diseases [40].
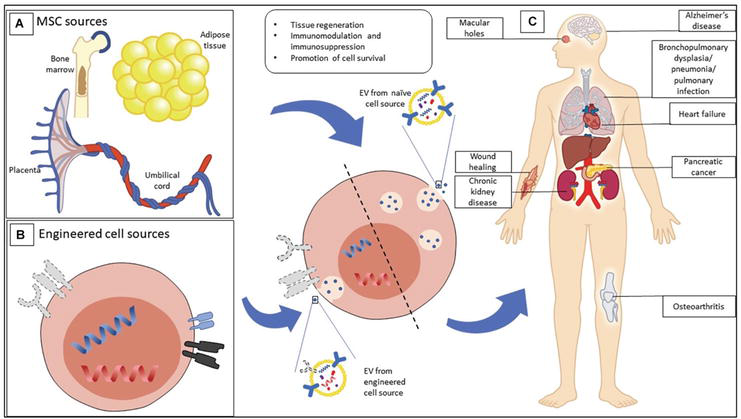
Figure 1.
Mesenchymal stromal cells (MSCs) EVs show therapeutic potential in a wide scale. (A) MSC-EVs had multiple isolation sources that include birth-associated tissues, adipose tissues, and bone marrow tissues. (B) MSCs are known to alter and import properties of demand for treatment. (C) EVs of both natural and engineered sources explored a wide range of clinical indicators. Adopted from: [
MSC-EVs are known to lodge therapeutic small molecules that promote regeneration in damaged tissues by lowering inflammation and inhibit apoptosis. MSCs have been recently engineered to enhance bone regeneration [41]. Another characteristic of EVs is to transfer non-immunogenic capsules that appeared to be ideal as drug vehicles, such as for tumor cells. This idea of EVs fastens new strategies to produce therapeutic cargo. These methods could be associated with the above incubation with drug delivery or transfecting of cells to allow specific small molecules into EVs. EV loading can also be facilitated after EV isolation from cell culture. Techniques including freeze-thawing, sonication, electroporation, osmotic shock, and saponin permeabilization have been adopted to temporarily disrupt the EV membrane sufficient for the uptake of therapeutic cargo.
6. Antiviral properties of MSCs
MSCs typically are virus-resistant cells compared to their more differentiated cell types. Such an ability was obtained by MSCs
Indoleamine 2,3 dioxygenase (IDO) nutrient deprivation is a useful antiviral MSC strategy, and this effect has been observed against measles virus, cytomegalovirus, herpes simplex virus-1, and HBV [25, 44]. IDO seems to be a fundamental antiviral molecule of the MSCs. Another antiviral mechanism by MSCs is the production of noncoding miRNAs with antiviral activity by targeting viral replication. For instance, the antiviral activity of MSCs against hepatitis C virus (HCV) is conferred by Let-7f, miR-145, miR-199a, and miR-221 in derived extracellular vesicles (EVs) [44].
7. MSC-based therapies for COVID-19
The outbreak of the pandemic novel coronavirus (COVID-19) during 2019 drastically increased the number of patients and mortality rate worldwide [45]. Although the vaccine has been developed, rapid mutations made the virus more complex, and therefore, effective treatment measures were challenging. To date, the benefits of MSC-based viral therapy working on the basis of its immunomodulation, antiviral, anti-apoptotic, anti-infective, and angiogenic properties are promising [46]. While using a cellular product, it cannot suppress patients when dealing with the infection or must not make it susceptible to other infections [46]. MSCs could overcome this scenario because of their ability to fight against virus infection with immunomodulatory and regenerative abilities [13, 47]. As a concern of available resources, the preclinical models of acute lung injury, acute respiratory distress syndrome (ARDS), viral hepatitis, human immunodeficiency virus (HIV) infection, and viral pneumonia have been evaluated in the last years [48, 49, 50]. To date, there are no standard protocols designed that improve the therapeutic potential of MSCs to target the viral attack [51, 52]. Therefore, much safety has ensured on MSC-based therapy on all clinical trials with small patient groups. Some efforts were made only to boost the antimicrobial activity of MSCs. Hypoxia priming of MSCs increases microvesicles to release growth factors, upregulate chemokine-receptors, and decrease cellular senescence and thus import therapeutic efficiency [52].
COVID-19 represents public health emergencies that urge the need of alternative therapy [29, 53, 54, 55]. Almost every COVID-19 victim experienced severe lung complications such as ARDS were observed only in some limited cases. SARS-CoV-2 is thought to be a major disease complication with COVID-19 with rise in mortality [56]. At this point, MSC-based therapy line would be plausible because of the easy biological properties of the MSCs that can be easily expanded when intravenously infused. Owing to their remarkable immunomodulatory and regenerative abilities, MSCs could attenuate the cytokine and prevent progression to ARDS and protect victims from multiple organ failure in severe COVID-19 condition [56]. As an advantage, the intravenously infused MSCs are trapped into the lungs, and this in fact marks beneficial as the lungs were the primary organ targets of SARS-CoV-2 [56, 57].
Attention has to be ensured that MSCs are not transfused during the initial period of viral infection. When wrongly used, the immunosuppression of MSCs hinders physiological and replication inflammation that are much essential to control viral infection [58]. Several other challenges have to be taken into context when MSCs are allowed in therapy line. The time and dosage of MSCs during administration must be calculated; if exceeded the exacerbated immunosuppression may have negative effect on the victim [56]. When administrated to large cohort groups, it must be ensured that the design plan is accurate and significant. Besides all of them, a good quality standard in the trials under appropriate regulatory supervision must be followed and has to be reported periodically in a complete and transparent manner. Ethical guidelines provided by the World Health Organization (WHO) for using cell-based therapy in clinical trials must be appropriate while using MSCs for COVID-19. Both ethical and moral aspects should be followed when performing the clinical trials during non-pandemic situation.
The critical pathological features of COVID-19 hospitalization are acute lung injuries (ALI) and ARDS characterized by immunopathological complications. Any treatment that hastens COVID-19 recovery would be in a substantial demand, and so, MSC therapeutics would be an ideal approach to handle the situation of COVID-19 symptoms due to their potential antiviral properties [59]. MSCs release various cellular components such as keratinocyte growth factor, prostaglandin E2, granulocyte-macrophage colony-stimulating factor (GM-CSF), IL-6, and IL-13 to facilitate phagocytosis and to further activate alveolar macrophages, alter the cytokine secretion profile of dendritic cell subsets, and decrease the limit of interferon γ from NK-cells. Tryptophan catabolizing enzyme indoleamine 2,3-dioxygenase is found to suppress T-cell proliferation, and this could even change the cytokine profile of T-cells. Furthermore, the proliferation and differentiation of B cells were notably impaired by MSCs as well. However, these effects were restored by the MSCs, and all these mentioned functions might also be worked out by MSCs in COVID-19 infections also. One among the potential outbursts of COVID-19 is the elevated levels of inflammatory cytokines due to overstimulation of immune cells resulting in a cytokine storm that eventually damaged the tissues and organs, especially the lungs. On the other hand, systematic treatment measures using MSCs have been increasing and a complete idea on their effect is still lacking. In this book chapter, the meta-analysis of COVID-19 retrieved from various sources is discussed.
8. Meta-analysis and systematic review
This review protocol was planned through a module in which the eligible criteria were defined according to the PICOS (population intervention, comparison, outcomes, and study) format. The target study population was all COVID-19 laboratories that confirmed patients received MSC treatment with an age group of 18 and above regardless of the gender. The disease severity from moderate to severe cases was involved. The experimental group was administrated with non-modified form of MSCs from BM and PTs (e.g., placenta, umbilical cord). Studied that used MSCs derived from embryonic stem cells (ESCs) and pluripotent stem cells (IPSC) were excluded. Genetically modified MSCs were also excluded. The reports were compared with the non-MSCs receiving group. The outcomes were represented such as groups with mortality and adverse effects (AEs), reduced inflammatory reactions based on the schematic markers, and third category of improvement in pulmonary function and oxygenation. Including the experimental outcomes, the article publications were retrieved from 6 databases (Figure 2) for analysis in this review that includes terms and limitations (Table 1).
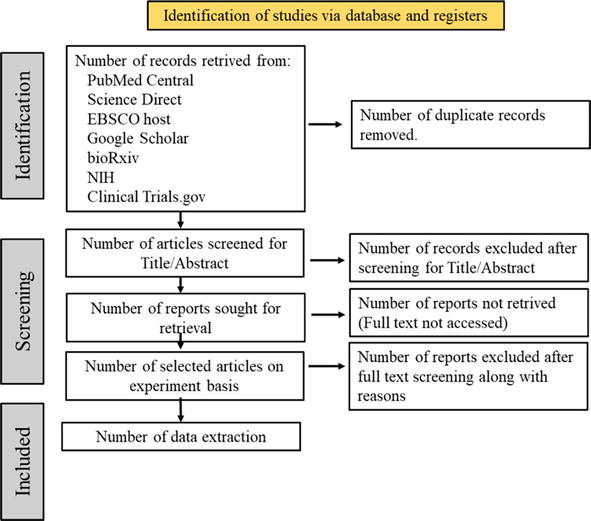
Figure 2.
PRISMA 2020 flow diagram shows data collection sources.
Databases | Search terms and limitations |
---|---|
PubMed Last searched: Nov 7, 2022 | Search terms: (Mesenchymal stem cells OR Mesenchymal stromal cells OR MSC) AND (COVID-19 OR SARS-CoV-2) Filter: The search results were filtered for clinical trials and randomized controlled trials only |
Science direct Last searched: Nov 7, 2022 | Search terms: Mesenchymal stem cells OR Mesenchymal stromal cells or MSCs and COVID-19 or SARS-CoV-2 and cytokine storm OR cytokine release syndrome Filters: The year of publication was set from 2020 to 2022 to remove irrelevant articles The results were filtered to generate research articles and case reports only |
EBSCO host Last searched: Nov 7, 2022 | Search terms: Mesenchymal stem cells OR Mesenchymal stromal cells OR MSC) AND (COVID-19 OR SARS-CoV-2) AND (cytokine storm OR cytokine release syndrome) Filters: Searches by Boolean/Phrase was applied Results were filtered for full text English articles The terms were searched within full text of the articles Search for equivalent subjects was applied The year of publication was set from 2020 to 2022 to remove irrelevant articles The source type was filtered to academic journals only |
Google scholar Last searched: Nov 15, 2022 | Search items: Mesenchymal stem cells OR Mesenchymal stromal cells or MSCs and COVID-19 or SARS-CoV-2 and cytokine storm OR cytokine release syndrome Filters: The year of publication was set from 2020 to 2022 to remove irrelevant articles The results were filtered to generate research articles and case reports only |
The Cochrane Library Last searched: Nov 7, 2022 | Search terms: (Mesenchymal stem cells OR Mesenchymal stromal cells OR MSC) AND (COVID-19 OR SARS-CoV-2) *No filter applied |
BioRxiv Last searched: Nov 15, 2022 | Search items: Mesenchymal stem cells OR Mesenchymal stromal cells or MSCs and COVID-19 or SARS-CoV-2 and cytokine storm OR cytokine release syndrome Filters: The year of publication was set from 2020 to 2022 to remove irrelevant articles |
ClinicalTrials.gov Last searched: Nov 7, 2022 | Search items: Mesenchymal stem cells OR Mesenchymal stromal cells or MSCs and COVID-19 or SARS-CoV-2 and cytokine storm OR cytokine release syndrome Filters: The year of publication was set from 2020 to 2022 to remove irrelevant articles |
Table 1.
* SARS-CoV, severe acute respiratory syndrome coronavirus 2.
Randomized controlled trial (RCT) was conducted for quality assessment with the incidence of mortality, AEs, and serious adverse effects (SAEs). The levels of CRP and IL-6 were assessed with the intention to treat the disease in an effective way. Meta-analysis on pulmonary function was not performed due to insufficient data. Of all the 100 articles retrieved, many articles failed in full-text retrieval, so 8 articles were selected finally for data extraction. Table 2 shows the characteristics of review, which includes the details of treatment used. The umbilical cord-derived MSCs were intravenously infused into the COVID-19 patients. The outcomes of the study that included the mortality occurrence and AEs, elevation in the inflammatory responses, and improvement in patient pulmonary were recorded and tabulated (Table 3). It is noteworthy that patients in the MSCs-treated group were reported with less mortality when compared with those in the control group [60, 61]. Various levels of inflammatory responses were studied by researchers. The inflammatory markers CRP and procalcitonin (PCT) and certain pro-inflammatory cytokines like IL-6, IL-2, tumor necrosis factor alpha (TNF-α), and anti-inflammatory cytokine (IL-10) were compared in the meta-analysis. Those with MSCs treated cases notably decreased in the cytokines were observed [62]. Studies led by Adas and co-workers [63] reported increased levels of anti-inflammatory cytokine IL-10. Similarly, another study showed higher expression of IL-10 in MSC-treated patients [61]. However, CT score of MSC-applicable groups showed improvement in the lung clearance, the number of lobes involved, and ground-glass opacity (GGO) [64, 65, 66].
Sources | Study types | Participants (n) | Mesenchymal stromal/stem cells | ||||
---|---|---|---|---|---|---|---|
Experimental group | Control group | Sources | Passage | Dose | Delivery method | ||
Adas et al. | Randomized, standard treatment- controlled trial, three parallel armed (two control arms) | Group 3 (critical illness): 10 | Group 1 (moderate illness): 10 Group 2 (critical illness): 10 | Wharton’s Jelly | 4 | 3 × 106 cells/kg in 150 ml of 0.9% NaCl (three infusions) | Intravenous infusion |
Dilogo et al. | Multicentered, double-blind, randomized, placebo-controlled trial | 20 | 20 | Umbilical cord | 5 or 6 | 1 × 106 cells/kg in 100 ml of 0.9% NaCl (single infusion) | Intravenous infusion |
Lanzoni et al. | Phase I/IIa, double-blind, randomized, placebo-controlled trial | 12 | 12 | Umbilical cord | N/A | 100 ± 20 × 106 cells/ infusion in 50 ml vehicle solution containing HSA and heparin (two infusions) | Intravenous infusion |
Liang et al. | Case report | 1 | 0 | Umbilical cord | 5 | 5 × 107 cells/infusion in 0.9% NaCl with 5% human albumin (three infusions) | Intravenous infusion |
Monsel et al. | Multicentered, double-blind, randomized, placebo- controlled trial | 21 | 24 | Umbilical cord | 4 | 1 × 106 cells/kg in 150 ml of 0.9% NaCl with 0.5% albumin (three infusions) | Intravenous infusion |
Rebelatto et al. | Phase I/II, prospective, single-centered, randomized, double-blind, placebo-controlled clinical trial | 11 | 6 | Umbilical cord | 3–5 | 5 × 105 cells/kg in 30 mL of vehicle solution containing saline solution, 5% anticoagulant citrate dextrose (ACD), and 20% albumin (three infusions) | Intravenous infusion |
Shu et al. | Single-centered open-label, individually randomized, standard treatment- controlled trial | 12 | 29 | Umbilical cord | 3–5 | 2 × 106 cells/kg in 100 ml of normal saline (single infusion) | Intravenous infusion |
Zhu et al. | Case report | 1 | 0 | Umbilical cord | N/A | 1 × 106 cells/kg in 100 ml of 0.9% NaCl (single infusion) | Intravenous infusion |
Table 2.
Study characteristics comprising the design and details of MSC treatment.
MSC, mesenchymal stromal/stem cell; HAS, human serum albumin; N/A, not available.
Sources | MSC-treated | Control | ||||||||
---|---|---|---|---|---|---|---|---|---|---|
Number of death events | Number of patients of with AEs patients with SAEs | Total Mortality rate (%) | Number of death events | Number of patients with AEs | Number of patients with SAEs | Total Mortality rate (%) | ||||
Adas et al. Turkey | 3 | N/A | N/A | 10 | 30 | 6 | N/A | N/A | 10 | 60 |
Dilogo et al. Indonesia | 10 | N/A | N/A | 20 | 50 | 16 | N/A | N/A | 20 | 80 |
Lanzoni et al. United States | 2 | 8 | 2 | 12 | 16.67 | 7 | 11 | 8 | 12 | 58.33 |
Liang et al. China *Case report | 0 | 0 | 0 | 1 | 0 | N/A | N/A | N/A | N/A | N/A |
Monsel et al. France | 5 | 18 | 6 | 21 | 23.81 | 4 | 18 | 6 | 24 | 16.67 |
Rebelatto et al. Brazil | 5 | N/A | N/A | 11 | 45.45 | 1 | N/A | N/A | 6 | 16.67 |
Shu et al. China | 0 | N/A | N/A | 12 | 0 | 3 | N/A | N/A | 29 | 10.34 |
Zhu et al. China *Case report | 0 | 0 | 0 | 1 | 0 | N/A | N/A | N/A | N/A | N/A |
Table 3.
Incidence of mortality and adverse events in MSC-treated and control groups.
AE, adverse event; N/A, not available; MSC, Mesenchymal stromal/stem cell; SAE, serious adverse event.
In this book review, it was observed that the efficacy of MSCs was determined based on the inflammatory markers and pulmonary function in the COVID-19 patients. Due to insufficient data on pulmonary function, the meta-analysis was not performed. Thus, for convenience, details of CPR and IL-6 were included in this meta-analysis to standardize the study results [61].
9. Main pathways of MSCs immunomodulation
The immunomodulatory function of MSCs is versatile and is widely described here. The establishment of response is defined as the important regulatory work of MSCs and immune responses. The iNOS-NO axis induced cytokines that are involved to mediate the immunoregulation of rodents such as mouse, rat, and hamster, whereas IDO is preferably useful for mammalian species [67]. Upon activation by pro-inflammatory cytokines, the murine MSCs produce a high level of iNOS and NO. Inhibition of iNOS abolishes the mouse MSC-mediated antiproliferative effect on T cells [68]. SH2 domain-containing phosphatase-1 (SHP1) negatively modulates the iNOS expression in MSCs. High level of JAK1 and STAT3 phosphorylation shall occur to produce more iNOS and cyclooxygenase 2 (COX2) when SHI1 is deficient in MSCs that result in more immunosuppressive liver injury. NO may coordinate with phosphorylated STAT3 to increase PD-L1 expression in IL-17-stimulated MSCs. Thus, MSCs with pre-treated IL-17 acquire high potent immunosuppressive capacity with modulated mRNA stability through degrading ARE/poly(U)-binding/degradation factor 1 (AUF1). NO shall therefore be lost through oxidation. In order to be effective, T cells have to be attached in close proximity to MSCs by chemokines by adhesion molecules such as ICAM-1 and VCAM-1. During tuberculosis progression, the pathogen recruits MSCs to the site and induces NO production, blunting T-cell responses and helping the bacterium to invade host immune responses [69]. Similar attempt was also noted with Coxsackievirus B3 (CVB3)-induced myocarditis, indicating MSCs to stimulate antiviral immunity to blunt T-cell activation in NO-dependent. Under inadequate stimulus or insufficient inflammation-exposure time, NO-mediated immunosuppression by MSCs is likely useful to enhance the effect. Ablation of iNOS expression in MSCs could still enhance immune response because chemokines attract immune cells and enhance immune response both
MSCs express Toll-like receptors (TLRs) to recognize pathogens including viruses [74, 75]. MSCs derived from adipose tissues express TLR2, TLR3, TLR4, and TLR9 through transcriptional and translational levels and provide TLR machinery to activate inflammatory NF- κB pathway and interferon factors, which are important for viral infection. MSC secretome helps the organism to repair damaged areas through secretions of proangiogenic, antiapoptotic, and antifibrotic factors. Due to severe inflammation and tissue destruction, the nutritional and oxygen depletion level is impaired that results in organic ischemia. Therefore, neo-vessels have to raise blood perfusion with maximized number of viable cells to finally restore tissue function and prevent tissue fibrosis [76]. Antiapoptotic factors secreted by MSC are ANG, ANGPT1, bFGF, CXCL12, EGF, ESM1, GF-1, IL-6, JAG1, LIF, MCP-1, MMP-1, PDGF, PIGF, PTN, STC1, TGF-β, and VEGF [77, 78].
10. MSCs attenuate COVID-19
One of the major problems that resulted during COVID-19 is multiple organ failure and death due to lung endothelial damage and activation of blood coagulation, which is also accompanied by cytokine storm. MSCs offer a promising innovative strategy for attenuating the cytokine storm and ultimately improving patient outcomes (Figure 3). Several methods of infusions (intravenous, intra-arterial, & direct) are in practice in which in the intravenous method, the MSCs get trapped into the inflamed lungs and exert immunomodulatory response directly by reacting with the epithelial and immune cells of the lungs. This response releases various mediators that ultimately reduce inflammation and protect epithelial cells in alveoli [79, 80, 81]. Administration through intratracheal in COVID-19 cases is also found to be conceivable and might work better. Recent studies have shown robust evidence with MSCs to treat lung injury and ARDS. Exposure of lung cells to MSCs results in reduced pro-inflammatory cytokines including IL-1α, IL-1β, IL-6, IFN-γ, and TNF-α and an increase in anti-inflammatory cytokines such as IL-4, IL-5, and IL-10, thus restoring the fluid clearance of the lungs, thickening alveoli, increasing air space volume, and reducing inflammation markers [82]. MSCs can inhibit platelet activation through CD73 ectonucleotidase activity, which is one of key MSC membrane markers. Hence, MSCs might play a crucial role in dampening both inflammation and hypercoagulopathy status during SARS-CoV-2-related severe pneumonia [83]. MSCs isolated from different sources largely differ in their incompatibility of the expression of tissue factor (TF). For instance, bone marrow MSCs (BM-MSCs), demonstrate a high level TF expression with reduced hemocompatibility [81].
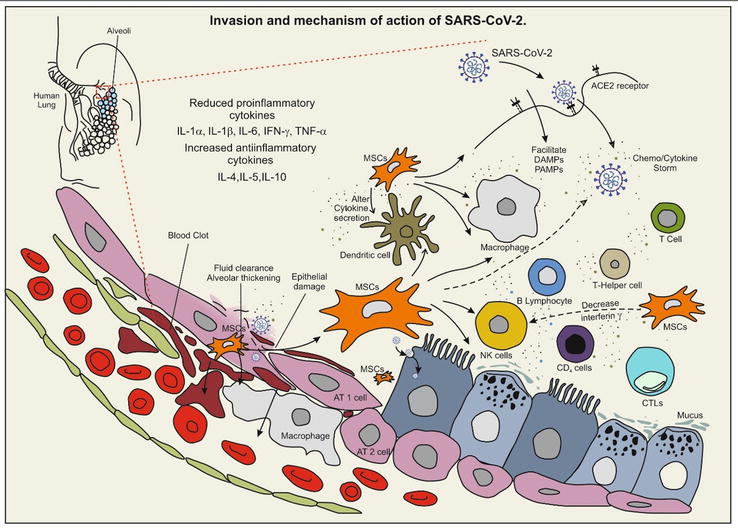
Figure 3.
Invasion and mechanism of action of SARS-CoV-2. The virus entry is facilitated through ACE2 cell surface receptor protein (angiotensin covering enzyme-II). Viral entry triggers the activation of immune response in dendritic cells, T-cells, B cells, macrophages, T-helper cells, CTLs, and NK cells. Chemokines/cytokine storm results in organ dysfunction and tissue damage in lung and alveolar cells. MSCs sources present in various body sites upon activation reduced damage by altering cytokine of dendritic cells and through decrease interferon-γ in NK-cells result relief outcome. Viral molecules facilitate cell rupture, fluid accumulation, alveolar thickening, and blood clot formation in AT1 and AT2 lung cells through damage-associated molecular patterns (DAMPs) and pathogen-associated molecular patterns (PAMPs), thus inducing MSC1 phenotype. Expressed interleukin IL-1α, IL-1β, and IL-6; interferon gamma (INF-γ) and TNF-α; and increase in anti-inflammatory cytokines such as IL-4, IL-5, and IL-10 help protect the lung cells; GM-CSF - granulocyte-macrophage Colony-stimulating factor, SARS-CoV-2 - severe acute respiratory syndrome Coronavirus-2, TNF - tumor necrosis factor, MSCs - mesenchymal stromal cells.
11. Anti-inflammation and immunomodulation COVID-19
Inflammatory modulation of MSCs is a key to the successful control of COVID-19. Corticosteroid therapy as it exerts potential anti-inflammatory effect was relatively restricted as it delays in virus clearance [84]. Thus, the urge of therapeutic interventions with anti-inflammatory effect was needed. MSCs are capable of reducing this risk, thereby protecting epithelial lung cells from undergoing death of COVID-19 [85]. Preclinical studies have showed MSCs could save acute alveolar injury in mouse model of ALI/ARDS [80, 86]. Once lodged into the lungs, MSCs secreted secretome (EVs) to exert anti-inflammatory effects. When incorporated into COIVD-19 patients, MSCs increased the peripheral lymphocytes with CRP levels decreased. Surprisingly, COVID-19 patients after MSC-based therapy displayed reduction in TNF-a level, a pro-inflammatory cytokine, and an increased anti-inflammatory mediator IL-10. The anti-inflammatory effect exerted by MSCs is a part of paracrine pathway. MSCs release anti-inflammatory cytokines and factors such as growth factor-b (TGFβ), vascular endothelial growth factor (HGF), vascular endothelial growth factor (VEGF), epidermal growth factor (EGF), and brain-derived neurotrophic factor (BDNF). MSC-derived secretomes (EVs) contain specific peptides that are migrated to the injured site and have an effect on the treatment of subsequent pulmonary fibrosis. After invasion of SAR-CoV-2 in the host environment stimulated innate and subsequently adaptive immune response. Antigen presenting cells (APCs) drove the virus-derived compounds to T cells and triggered immune response. Though the antiviral response is much essential for the virus clearance, damages to the alveolar epithelium and lung endothelium have to be repaired [87]. During viral destruction, the immune system is highly activated with high levels of inflammatory cytokines worsening the lung injury and organ damage. MSCs with immunomodulatory property inhibit the innate immune response as well as adaptive responses [88]. MSCs therefore regulate the activity of T and B cells, macrophages, monocytes, dendritic cells, and NK cells through cell–cell communication and through secretome [80]. MSCs have been found to exert immunosuppressive functions
12. MSC-based therapy of viral hepatitis
Experimental studies have documented the beneficial effect of attenuation of acute hepatitis and liver failure. MSCs were observed to suppress the activation of hepatotoxic IFN-γ and IL-17 in an NO- and IDO-dependent manner. This expression produces numerous cytokines and induces proliferation of FoxP3 and NKT cells. Additionally, MSCs promote hepatocyte proliferation and liver regeneration. The BM-derived MSCs helped survive 56 acute-on-chronic liver failure (ACLF) patients from hepatitis B virus. For this, MSCs were injected intravenously once in a week for 4 weeks. No infusion-related adverse effect was noted, which indicated the safety of MSCs in disease treatment for ACLF. As laboratory measurements, the total serum bilirubin for patients with End-Stage Liver Disease (MELD) score treated with MSCs was compared with a control group where normal treatment was acquired [91]. The infection was higher in the group of patients who received standard therapy than in the MSC-treated group. MSCs manage to suppress the activation of hepatotoxic immune cells without changing systemic immunosuppression and immunodeficiency [92]. Similar to this observation, a single stage umbilical cord-derived MSC was noted to improve the liver function of patients treated with HBV-ACLF. The improvement was ensured by hepatocyte-functioning markers including, albumin, alanine aminotransferase, aspartate aminotransferase, total bilirubin, prothrombin time (PT), and international normalized ratio (INR). A significant reduction in MELD score was noticed in patients after 4 weeks without causing side effects considering MSCs as an adjuvant therapy to treat HBV-ACLF. UC-MSC exosomes (UC-MSC-Exos) significantly improved the therapeutic efficacy of IFN-α, which are used as a standard therapy for patients with hepatitis C virus (HCV) infection. UC exosomes contain numerous immunosuppressive and miRNAs that bind to HCV RNA and prevent its replication.
13. Neutrophil extracellular traps (NETs) in COVID-19
During the severity of COVID-19 viral infection, networks of DNA-bound histone molecules wrapped around and detains the viral infection were observed. This sophisticated web-like structure called NETs through NETosis liberated by neutrophils upon activation was studied [93]. It was believed that NET production was triggered through fungal and bacterial infection. However, during COVID attack, it was reported that NETosis expression could defend viral diseases [94]. NETosis is known to be a cell-controlled process, but its mechanism still remains a mystery with some proven evidences stating that its expression begins in an ROS-independent manner [95]. Upon activation of neutrophils through viral infection, the nuclear envelop disintegrates, enabling the DNA to mix with granular proteins that are lines with effector proteins and peptidyl arginine deiminase type IV (PAD4) activation [95]. Proteins including neutrophil elastase (NE) and myeloper oxidase (MPO) stimulate chromatin condensation and deteriorate histones [96]. In the presence of histone hypercitrullination, PAD4 regulates decondensation and DNA-protein complexes are released extracellularly as NETs [97]. During chronic obstructive pulmonary disease and in ARDS patients, the levels of NETs were increased [98].
Nicolai et al. [99] and Skendros et al. [100] found the NET-related fibrin and platelet aggregation in COVID-19 patients and in SARS-CoV-2 infection alter the disease severity. Similar studies conducted by Middleton et al. [101] found elevated NET formation with COVID-19-related ARDS. The release of NETs during viral infection seems to change the NE production that has changes in the macrophage role by cleavage of TLRs. In addition, cytokines TNF-α and IL-8 can lead to the increase in neutrophil release of NETs. In a study led by Veras
Increased levels of cell-free DNA, myeloperoxidase-DNA (MPO-DNA) and citrullinated histone H3 (Cit-H3) were reported in the sera of COVID-19 patients [103]. Although the literature does not directly support the action mechanism of NETs with viral diseases, NETs found in sites of viral infection entrapping virus particles in a DNA web such that of SARS-CoV-2, influenza, COVID-19, and syncytial respiratory viral infection were reported [49, 50]. Therefore, treatments using the NETs to the new COVID-19 could reduce the disease severity caused by hyperinflammation [104], avoiding invasive mechanical ventilation and hence reduceing the mortality rate. Drugs such as gasdermin D [105], PAD4 [106], and NE [107] are known inhibitors that block molecules necessary for NET synthesis. Treatments on NETs in COVID-19 patients using various NET inhibitors are still in the development stage. Therefore, in the sense of COVID-19, treatments using NET-targets reduce the damage caused to lung cells and hyperinflammation, and research has to be considered further in various aspects to underline the neutrophil response mechanism and NETosis.
14. MSCs in the treatment of SARS-CoV-2
SARS-CoV-2-induced infection affects pneumocytes and ciliated cells of the lungs and results in alveolar injury and lung inflammation. In many cases of COVID-19 patients, the effective elimination of virus happens by activated alveolar macrophages, DCs, and T cells. Due to the overaccumulation of inflammatory cytokines and highly activated immune cells, cytokine storm occurs in some patients, making it complicated, which leads to life-threatening pneumonia, lung edema, and acute respiratory distress syndrome (ARDS) [85]. MSC-sourced hepatocyte growth factor (HGF), IL-10, and TGF-β act synergistically to induce alternatively activated anti-inflammatory (M2) phenotypes in alveolar macrophages. MSCs directly suppress the expansion of inflammatory IFN-γ producing Th1 and IL-17 in the injured lungs [47, 108]. MSCs, on the other hand, induce program death ligand (PDL) to induce apoptosis in over-activated T-cells. In addition, MSC-sourced TGF- β and HGF cause G1 cell cycle arrest, thereby suppressing the activation of JAk–Stat signaling pathway. Since MSCs effectively suppress immune response and provide additional oxygen supply to the injured lungs, investigations on the therapeutic potential of MSCs have proven to be significant. Within 48–96 h after MSC infusion in patients with SARS-CoV-2, the oxygen saturation level significantly increased and pneumonia-related symptoms of shortness of breath, cough, and fever started to disappear. It was confirmed by a computer tomography (CT) [109]. More importantly, MSCs prevented the influx of inflammatory immune cells in the patient’s lungs, favored the expansion of anti-inflammatory cells, restored the function of liver and kidney, and prevented multiple organ dysfunctions.
15. Conclusion and future perspectives
Mesenchymal stem cells (MSCs) are considered to be a promising therapeutic (Figure 1) method of more severe viral infections like COVID-19. Due to their prominent mechanism in action (Figure 2) to various levels and demonstrated safety profile in the early phase studies, MSCs have been a major research focus in the recent years and in phase 2 clinical trials of COVID-19 pneumonia. In the context of well-studied differentiation potentials and immunomodulatory properties of MSCs are appealing to treat immunological disorders. Understanding more on the plasticity of MSC-mediated immunoregulation will help to guide the appropriate potential of MSCs. Another important thing to be considered is the pathophysiological role of MSCs in their original and inflammatory form. Although MSCs are involved to treat various immunological disorders, the role of tissue-resident in immunomodulation yet needs to be more investigated. Furthermore, new markers should be identified for specific inflammatory condition and MSC-based clinical protocols to be optimized so as to respond at different levels of disease progression.
References
- 1.
Pandey A, Khan MK, Hamurcu M, Gezgin S. Natural plant products: A less focused aspect for the COVID-19 viral outbreak. Frontiers in Plant Science. 2020; 2020 (11):568890 - 2.
Harris G, Adalja A. ICU preparedness in pandemics: Lessons learned from the coronavirus disease outbreak. Current Opinion in Pulmonary Medicine. 2019; 27 :73-78 - 3.
Rocha JLM, de Oliveira WCF, Noronha NC, dos Santos NCD, Covas DT, Picanço-Castro V, et al. Mesenchymal stromal cells in viral infections: Implications for COVID-19. Stem Cell Reviews and Reports. 2021; 17 :71-93 - 4.
Ahmadi AR, Chicco M, Huang J, Qi L, Burdick J, Williams GM, et al. Stem cells in burn wound healing: A systematic review of the literature. Burns. 2019; 45 :1014-1023 - 5.
Gazdic M, Volarevic V, Arsenijevic N, Stojkovic M. Mesenchymal stem cells: A friend or foe in immune-mediated diseases. Stem Cell Reviews and Reports. 2015; 11 :280-287 - 6.
Martin I, Galipeau J, Kessler C, Le Blanc K, Dazzi F. Challenges for mesenchymal stromal cell therapies. Science Translational Medicine. 2019; 11 (eaat2189):1-3 - 7.
Khoury M, Cuenca J, Cruz FF, Figueroa FE, Rocco PRM, Weiss DJ. Current status of cell-based therapies for respiratory virus infections: Applicability to COVID-19. The European Respiratory Journal. 2020; 55 :2000858. DOI: 10.1183/13993003.00858-2020 - 8.
Volarevic V, Gazdic M, Simovic Markovic B, Jovicic N, Djonov V, Arsenijevic N. Mesenchymal stem cell-derived factors: Immuno-modulatory effects and therapeutic potential. BioFactors. 2017; 43 :633-644 - 9.
Thanunchai M, Hongeng S, Thitithanyanont A. Mesenchymal stromal cells and viral infection. Stem Cells International. 2015; 2015 :860950 - 10.
Avanzi S, Leoni V, Rotola A, Alviano F, Solimando L, Lanzoni G, et al. Susceptibility of human placenta derived mesenchymal stromal/stem cells to human herpesviruses infection. PLoS One. 2013; 8 (8):e71412-e71412 - 11.
Behzadi Fard M, Kaviani S, Atashi A. Parvovirus B19 infection in human bone marrow mesenchymal stem cells affects gene expression of IL-6 and TNF-α and also affects hematopoietic stem cells differentiation. Indian Journal of Hematology and Blood Transfusion. 2019; 35 (4):765-772 - 12.
Arzi B, Kol A, Murphy B, Walker NJ, Wood JA, Clark K, et al. Feline foamy virus adversely affects feline mesenchymal stem cell culture and expansion: Implications for animal model development. Stem Cells and Development. 2015; 24 (7):814-823 - 13.
Shi Y, Wang Y, Li Q, Liu K, Hou J, Shao C, et al. Immunoregulatory mechanisms of mesenchymal stem and stromal cells in inflammatory diseases. Nature Reviews Nephrology. 2018; 14 :493-507 - 14.
Naji A, Eitoku M, Favier B, Deschaseaux F, Rouas-Freiss N, Suganuma N. Biological functions of mesenchymal stem cells and clinical implications. Cellular and Molecular Life Sciences. 2019; 76 :3323-3348 - 15.
Leyendecker_Junior, A., Pinheiro, C., Amano, M, T., & Bueno, D. The use of human mesenchymal stem cells as therapeutic agents for the in vivo treatment of immune-related diseases: A systematic review. Frontiers in Immunology. 2018; 9 :2056. DOI: 10.3389/fimmu.2018.02056 - 16.
Vasandan AB, Jahnavi S, Shashank C, Prasad P, Kumar A, Jyothi Prasanna S. Human mesenchymal stem cells program macrophage plasticity by altering their metabolic status via a PGE2-dependent mechanism. Scientific Reports. 2016; 6 :38308. DOI: 10.1038/srep38308 - 17.
Longhi S. Structural disorder in viral proteins. Protein and Peptide Letters. 2010; 17 (8):930-931 - 18.
Roy E, Shi W, Duan B, Reid SP. Chikungunya virus infection impairs osteogenic differentiation of bone marrow-derived mesenchymal stem cells. mSphere. 2020; 5 :e00347-e00320. DOI: 10.1128/mSphere.00347-20 - 19.
De La Garza-Rodea AS, Verweij MC, Boersma H, van der Velde-van DI, de Vries AAF, Hoeben RC, et al. Exploitation of herpesvirus immune evasion strategies to modify the immunogenicity of human mesenchymal stem cell transplants. PLoS One. 2011; 6 (1):e14493-e14493 - 20.
Meisel R, Brockers S, Heseler K, Degistirici O, Bulle H, Woite C, et al. Human but not murine multipotent mesenchymal stromal cells exhibit broad-spectrum antimicrobial effector function mediated by indoleamine 2,3-dioxygenase. Leukemia. 2011; 25 (4):648-654 - 21.
Meisel R, Heseler K, Nau J, Schmidt SK, Leineweber M, Pudelko S, et al. Cytomegalovirus infection impairs immunosuppressive and antimicrobial effector functions of human multipotent mesenchymal stromal cells. Mediators of Inflammation. 2014; 2014 :898630 - 22.
Wu X, Dao Thi VL, Huang Y, Billerbeck E, Saha D, Hoffmann H-H, et al. Intrinsic immunity shapes viral resistance of stem cells. Cell. 2018; 172 (3):423-438.e425 - 23.
Meisel R, Zibert A, Laryea M, Göbel U, Däubener W, Dilloo D. Human bone marrow stromal cells inhibit allogeneic T-cell responses by indoleamine 2,3-dioxygenase-mediated tryptophan degradation. Blood. 2004; 103 (12):4619-4621 - 24.
Li Y, Xu J, Shi W, Chen C, Shao Y, Zhu L, et al. Mesenchymal stromal cell treatment prevents H9N2 avian influenza virus-induced acute lung injury in mice. Stem Cell Research & Therapy. 2016; 7 (1):159 - 25.
Qian X, Xu C, Fang S, Zhao P, Wang Y, Liu H, et al. Exosomal MicroRNAs derived from umbilical mesenchymal stem cells inhibit hepatitis C virus infection. Stem Cells Translational Medicine. 2016; 5 (9):1190-1203 - 26.
Loy H, Kuok DIT, Hui KPY, Choi MHL, Yuen W, Nicholls JM, et al. Therapeutic implications of human umbilical cord mesenchymal stromal cells in attenuating influenza A(H5N1) virus-associated acute lung injury. The Journal of Infectious Diseases. 2019; 219 (2):186-196 - 27.
Spaggiari GM, Capobianco A, Becchetti S, Mingari MC, Moretta L. Mesenchymal stem cell-natural killer cell interactions: Evidence that activated NK cells are capable of killing MSCs, whereas MSCs can inhibit IL-2-induced NK-cell proliferation. Blood. 2006; 107 (4):1484-1490 - 28.
Spaggiari GM, Capobianco A, Abdelrazik H, Becchetti F, Mingari MC, Moretta L. Mesenchymal stem cell inhibit natural killer–cell proliferation, cytotoxicity, and cytokine production: Role of indoleamine 2,3-dioxygenase and prostaglandin E2. Blood. 2008; 111 (3):1327-1333 - 29.
Zhou M, Zhang X, Qu J. Coronavirus disease 2019 (COVID-19): A clinical update. Frontiers of Medicine. 2020; 14 :126-135 - 30.
Karlsson H, Samarasinghe S, Ball LM, Sundberg B, Lankester AC, Dazzi F, et al. Mesenchymal stem cells exert differential effects on alloantigen and virus-specific T-cell responses. Blood. 2008; 112 (3):532-541 - 31.
Kang HS, Habib M, Chan J, Abavana C, Potian JA, Ponzio NM, et al. A paradoxical role for IFN-gamma in the immune properties of mesenchymal stem cells during viral challenge. Experimental Hematology. 2005; 33 (7):796-803 - 32.
Ren G, Zhang L, Zhao X, Xu G, Zhang Y, Roberts AI, et al. Mesenchymal stem cell-mediated immunosuppression occurs via concerted action of chemokines and nitric oxide. Cell Stem Cell. 2008; 2 (2):141-150 - 33.
Wiklander OP, Nordin JZ, O’Loughlin A, Gustafsson Y, Corso G, Mäger I, et al. Extracellular vesicle in vivo biodistribution is determined by cell source, route of administration and targeting. Journal of Extracellular Vesicles. 2015; 4 :26316 - 34.
Shelke GV, Lässer C, Gho YS, Lötvall J. Importance of exosomes depletion protocols to eliminate functional and RNA-containing extracellular vesicles from fetal bovine serum. Journal of Extracellular Vesicles. 2018; 3 :24783 - 35.
Lewis ND, Sia CL, Kirwin K, Haupt S, Mahimkar G, Zi T, et al. Exosome surface display of IL12 results in tumor-retained pharmacology with superior potency and limited systemic exposure compared with recombinant IL12. Molecular Cancer Therapeutics. 2021; 20 :523-534 - 36.
Lydia AE, Yiqi S, Hai Fang Y, Corinne B, Samira L, Matthew JAW. Delivery of siRNA to the mouse brain by systemic injection of targeted exosomes. Nature Biotechnology. 2011; 29 :341 - 37.
Johnson J, Shojaee M, Mitchell Crow J, Khanabdali R. Mesenchymal stromal cells to engineered extracellular vesicles. A new therapeutic paradigm. Frontiers in Cell and Developmental Biology. 2021; 9 :705676 - 38.
Tian T, Cao L, He C, Ye Q, Liang R, You W, et al. Targeted delivery of neural progenitor cell-derived extracellular vesicles for anti-inflammation after cerebral ischemia. Theranostics. 2021; 11 :6507-6521 - 39.
Zhilan Y, Tao Z, Wenshan H, Honglin J, Cuiwei L, Zhe Y, et al. Methotrexate-loaded extracellular vesicles functionalized with therapeutic and targeted peptides for the treatment of glioblastoma multiforme. ACS Applied Materials & Interfaces. 2018; 10 :12341 - 40.
Conceição M, Forcina L, Wiklander OPB, Gupta D, Nordin JZ, Vrellaku B, et al. Engineered extracellular vesicle decoy receptor-mediated modulation of the IL6 trans-signaling pathway in muscle. Biomaterials. 2021; 266 :120435 - 41.
Chun-Chieh H, Miya K, Yu L, Sajjad S, Iriarte-Diaz J, Lyndon FC, et al. Functionally engineered extracellular vesicles improve bone regeneration. Acta Biomaterialia. 2020; 109 :182 - 42.
Schoggins JW. Interferon-stimulated genes: What do they all do? Annual Review of Virology. 2019; 6 (1):567-584 - 43.
Kane M, Zang TM, Rihn SJ, Zhang F, Kueck T, Alim M, et al. Identification of interferon-stimulated genes with antiretroviral activity. Cell Host and Microbe. 2016; 20 (3):392-405 - 44.
Stebbing J, Phelan A, Griffin I, Tucker C, Oechsle O, Smith D, et al. COVID-19: Combining antiviral and anti-inflammatory treatments. The Lancet Infectious Diseases. 2020; 20 (4):400-402 - 45.
Moll G, Drzeniek N, Kamhieh-Milz J, Geissler S, Volk HD, Reinke P. MSC therapies for COVID-19: Importance of patient coagulopathy, thromboprophylaxis, cell product quality and mode of delivery for treatment safety and efficacy. Frontiers in Immunology. 2020; 11 :1091 - 46.
Wang Y, Chen X, Cao W, Shi Y. Plasticity of mesenchymal stem cells in immunomodulation: Pathological and therapeutic implications. Nature Immunology. 2014; 15 (11):1009-1016 - 47.
Harrell CR, Sadikot R, Pascual J, Fellabaum C, Jankovic MG, Jovicik Jovicic N, et al. Mesenchymal stem cell-based therapy of inflammatory lung diseases: Current understanding and future perspectives. Stem Cells International. 2019; 4236973 :1-15. DOI: 10.1155/2019/4236973 - 48.
Behnke J, Kremer S, Shahzad T, Chao C-M, Böttcher-Friebertshäuser E, Morty RE, et al. MSC based therapies—New perspectives for the injured lung. Journal of Clinical Medicine. 2020; 9 (3):682 - 49.
Qin H, Zhao A. Mesenchymal stem cell therapy for acute respiratory distress syndrome: From basic to clinics. Protein & Cell. 2020; 11 (10):707-722. DOI: 10.1007/s13238-020-00738-2 - 50.
Kouroupis D, Sanjurjo-Rodriguez C, Jones E, Correa D. Mesenchymal stem cell functionalization for enhanced therapeutic applications. Tissue Engineering - Part B: Reviews. 2019; 25 :55-77 - 51.
Noronha N, de Mizukami CA, Caliári-Oliveira C, Cominal JG, JLM R, Covas DT, et al. Correction to: Priming approaches to improve the efficacy of mesenchymal stromal cell-based therapies. Stem Cell Research & Therapy. 2019; 10 (1):132 - 52.
Yen BL, Yen ML, Wang LT, Liu KJ, Sytwu HK. Current status of mesenchymal stem cell therapy for immune/inflammatory lung disorders: Gleaning insights for possible use in COVID-19. Stem Cells Translational Medicine. 2020; 9 :1162-1173. DOI: 10.1002/sctm.20-0186 - 53.
Zhao RC. Stem cell-based therapy for coronavirus disease 2019. Stem Cells and Development. 2020; 29 (11):679-681 - 54.
Yu F, Jia R, Tang Y, Liu J, Wei B. SARS-CoV-2 infection and stem cells: Interaction and intervention. Stem Cell Research. 2020; 46 :101859 - 55.
Du J, Li H, Lian J, Zhu X, Qiao L, Lin J. Stem cell therapy: A potential approach for treatment of influenza virus and coronavirus-induced acute lung injury. Stem Cell Research and Therapy. 2020; 11 :192 - 56.
Chen X, Shan Y, Wen Y, Sun J, Du H. Mesenchymal stem cell therapy in severe COVID-19: A retrospective study of short-term treatment efficacy and side effects. Journal of Infection. 2020; 116 :1097-1100 - 57.
Golchin A, Seyedjafari E, Ardeshirylajimi A. Mesenchymal stem cell therapy for COVID-19: Present or future. Stem Cell Reviews and Reports. 2020; 16 :427-433 - 58.
World Health Organization. Managing Ethical Issues in Infectious Disease Outbreaks. Geneva, Switzerland: WHO; 2020. ISBN 978 9241549837, (NML classification: WA 105) - 59.
Su J, Chen X, Huang Y, Li L, Li J, Cao K, et al. Phylogenetic distinction of iNOS and IDO function in mesenchymal stem cell-mediated immunosuppression in mammalian species. Cell Death and Differentiation. 2014; 21 :388-396. DOI: 10.1038/cdd.2013.149 - 60.
Monsel A, Hauw-Berlemont C, Mebarki M, Heming N, Mayaux J, Tchoumba ON, et al. Treatment of COVID-19-associated ARDS with mesenchymal stromal cells: A multicentre randomized double-blind trial. Critical Care. 2022; 26 (1):48. DOI: 10.1186/s13054-022-03930-4 - 61.
Carmen L, Kuniyoshi R, Alexandra C, Claudio S, Franck L, Regina D, et al. Safety and long-term improvement of mesenchymal stromal cell infusion in critically COVID-19 patients: A randomized clinical trial. Stem Cell Research & Therapy. 2022; 13 :122 - 62.
Hadisoebroto I, Dita D, Adhrie A, Erlina S, Triya B, Pompini D, et al. Umbilical cord mesenchymal stromal cells as critical COVID-19 adjuvant therapy: A randomized controlled trial. Stem Cells Translational Medicine. 2021; 10 (9):1279-1287. DOI: 10.1002/sctm.21-0046 - 63.
Adas G, Cukurova Z, Kart Y, Yilmaz K, Isiksacan R, Kasapoglu N, et al. The systematic effect of mesenchymal stem cell therapy in critical COVID-19 patients: A prospective double controlled trial. Cell Transplantation. 2021; 30 :1-14. DOI: 10.1177/09636897211024942 - 64.
Bing L, Junhui C, Tao L, Haiying W, Wenjie Y, Yanjiao L, et al. Clinical remission of a critically ill COVID-19 patient treated by human umbilical cord mesenchymal stem cells a case report. Medicine (Baltimore). 2020; 99 :e21429. DOI: 10.1097/MD.0000000000021429 - 65.
Lei S, Changming N, Ruyou L, Tingrong H, Yan W, Mao H, et al. Treatment of severe COVID-19 with human umbilical cord mesenchymal stem cells. Stem Cell Research & Therapy. 2020; 11 :361. DOI: 10.1186/s13287-020-01875-5 - 66.
Yue Z, Rongjia Z, Kun L, Xin L, Dezhong C, Dunyao B, et al. Human umbilical cord mesenchymal stem cells for adjuvant treatment of a critically ill COVID-19 patient: A case report. Infection and Drug Resistance. 2020; 28 (13):3295-3300 - 67.
Kazuya S, Katsutoshi O, Iekuni O, Akiko M, Keiko H, Tadashi N, et al. Nitric oxide plays a critical role in suppression of T-cell proliferation by mesenchymal stem cells. Blood. 2007; 109 :228-234. DOI: 10.1182/blood-2006-02-002246 - 68.
Li W, Ren G, Huang Y, Su Y, Han Y, Li J, et al. Mesenchymal stem cells: A double-edged sword in regulating immune responses. Cell Death and Differentiation. 2012; 19 :1505-1513. DOI: 10.1038/cdd.2012.26 - 69.
Raghuvanshi S, Sharma P, Singh S, Van Kaer L, Das G. Mycobacterium tuberculosis evades host immunity by recruiting mesenchymal stem cells. Proceedings of the National Academy of Sciences of the United States of America. 2010; 107 :21653-21658 - 70.
Metghalchi S, Padmapriya P, Tabassome S, Alain T, Soraya T, Ziad M. Indoleamine 2,3-dioxygenase fine-tunes immune homeostasis in atherosclerosis and colitis through repression of interleukin-10 production. Cell Metabolism. 2015; 22 :460-471. DOI: 10.1016/j.cmet.2015.07.004 - 71.
Chen X, Gan Y, Li W, Su J, Zhang Y, Huang Y, et al. The interaction between mesenchymal stem cells and steroids during inflammation. Cell Death and Disease. 2014; 5 :e1009. DOI: 10.1038/cddis.2013.537 - 72.
Jitschin R, Bottcher M, Saul D, Lukassen S, Bruns H, Loschinski R, et al. Inflammation-induced glycolytic switch controls suppressivity of mesenchymal stem cells via STAT1 glycosylation. Leukemia. 2019; 33 :1783-1796. DOI: 10.1038/s41375-018-0376-6 - 73.
Song N, Wakimoto H, Rossignoli F, Bhere D, Ciccocioppo R, Chen K-S, et al. Mesenchymal stem cell immunomodulation: In pursuit of controlling COVID-19 related cytokine storm. Stem Cells. 2021; 39 (6):707-722 - 74.
Luchetti F. Melatonin regulates mesenchymal stem cell differentiation: A review. Journal of Pineal Research. 2014; 56 :382-397 - 75.
Zhong Y-S, Lin N, Deng M-H, Zhang F-C, Tang Z-F, Xu R-Y. Deficient proliferation of bone marrow-derived mesenchymal stem cells in patients with chronic hepatitis b viral infections and cirrhosis of the liver. Digestive Diseases and Sciences. 2010; 55 (2):438-445 - 76.
Lin YP, Shaw M, Gregory V, et al. Avian-to-human transmission of H9N2 subtype influenza A viruses: Relationship between H9N2 and H5N1 human isolates. Proceedings of the National Academy of Sciences of the United States of America. 2000; 97 (17):9654-9658 - 77.
Pak J, Chang J-J, Lee JH, Lee SH. Safety reporting on implantation of autologous adipose tissue-derived stem cells with platelet-rich plasma into human articular joints. BMC Musculoskeletal Disorders. 2013; 14 :337 - 78.
Blennow O, Fjaertoft G, Winiarski J, Ljungman P, Mattsson J, Remberger M. Varicella-zoster reactivation after allogeneic stem cell transplantation without routine prophylaxis—The incidence remains high. Biology of Blood and Marrow Transplantation. 2014; 20 (10):1646-1649 - 79.
Li Y, Chi Y, Bian Q, Wen T, Zhang W. Mesenchymal stem cells therapy for H9N2 avian influenza viruses induced acute lung injury in mice. In: Proceedings of the International Conference on Biomedical Engineering and Biotechnology (ICBEB’12). Macau, China: IEEE; 2012. pp. 1052-1055 - 80.
Ji F, Li L, Li Z, Jin Y, Liu W. Mesenchymal stem cells as a potential treatment for critically ill patients with coronavirus disease 2019. Stem Cells Translational Medicine. 2020; 9 :813-814 - 81.
Johnson CL, Soeder Y, Dahlke MH. Concise review: Mesenchymal stromal cell-based approaches for the treatment of acute respiratory distress and sepsis syndromes. Stem Cells Translational Medicine. 2017; 6 (4):1141-1151 - 82.
Islam MN, Das SR, Emin MT, et al. Mitochondrial transfer from bone-marrow-derived stromal cells to pulmonary alveoli protects against acute lung injury. Nature Medicine. 2012; 18 (5):759-765 - 83.
Dominici M, Le Blanc K, Mueller I, et al. Minimal criteria for defining multipotent mesenchymal stromal cells. The International Society for Cellular Therapy position statement. Cytotherapy. 2006; 8 (4):315-317 - 84.
Tatsumi K, Ohashi K, Matsubara Y, et al. Tissue factor triggers procoagulation in transplanted mesenchymal stem cells leading to thromboembolism. Biochemical and Biophysical Research Communications. 2013; 431 (2):203-209 - 85.
Lin BL, Chen JF, Qiu WH, Wang KW, Xie DY, Chen XY, et al. Allogeneic bone marrow-derived mesenchymal stromal cells for hepatitis B virus-related acute-on-chronic liver failure: A randomized controlled trial. Hepatology. 2017; 66 :209-219 - 86.
Zhang H, Baker A. Recombinant human ACE2: Acing out angiotensin II in ARDS therapy. Critical Care. 2017; 21 :305 - 87.
Zhang S, Jiang W, Ma L, Liu Y, Zhang X, Wang S. Nrf2 transfection enhances the efficacy of human amniotic mesenchymal stem cells to repair lung injury induced by lipopolysaccharide. Journal of Cellular Biochemistry. 2018; 119 :1627-1636 - 88.
Herold S, Becker C, Ridge KM, Budinger GR. Influenza virus induced lung injury: Pathogenesis and implications for treatment. The European Respiratory Journal. 2015; 45 :1463-1478 - 89.
Hosseinikia R, Nikbakht MR, Moghaddam AA, Tajehmiri A, Hosseinikia M, Oubari F, et al. Molecular and cellular interactions of allogenic and autologus mesenchymal stem cells with innate and acquired immunity and their role in regenerative medicine. International Journal of Hematology-Oncology and Stem Cell Research. 2017; 11 :63-77 - 90.
Li N, Hua J. Interactions between mesenchymal stem cells and the immune system. Cellular and Molecular Life Sciences. 2017; 74 :2345-2360 - 91.
Rashedi I, Gómez-Aristizábal A, Wang XH, Viswanathan S, Keating A. TLR3 or TLR4 activation enhances mesenchymal stromal cell-mediated treg induction via notch signaling. Stem Cells. 2017; 35 :265-275 - 92.
Sava RI, Pepine CJ, March KL. Immune dysregulation in HFpEF: A target for mesenchymal stem/stromal cell therapy. Journal of Clinical Medicine. 2020; 9 :241 - 93.
Jenne CN, Wong CHY, Zemp FJ, McDonald B, Rahman MM, Forsyth PA, et al. Neutrophils recruited to sites of infection protect from virus challenge by releasing neutrophil extracellular traps. Cell Host and Microbe. 2013; 13 (2):169-180 - 94.
Saitoh T, Komano J, Saitoh Y, et al. Neutrophil extracellular traps mediate a host defense response to human immunodeficiency virus-1. Cell Host & Microbe. 2012; 12 (1):109-116 - 95.
Yousefi S, Stojkov D, Germic N, Simon D, Wang X, et al. Untangling “NETosis” from NETs. European Journal of Immunology. 2019; 49 (2):221-227. DOI: 10.1002/eji.201747053 - 96.
Papayannopoulos V, Metzler KD, Hakkim A, Zychlinsky A. Neutrophil elastase and myeloperoxidase regulate the formation of neutrophil extracellular traps. The Journal of Cell Biology. 2010; 191 (3):677-691 - 97.
Fuchs TA, Abed U, Goosmann C, Hurwitz R, Schulze I, et al. Novel cell death program leads to neutrophil extracellular traps. The Journal of Cell Biology. 2007; 176 (2):231-241 - 98.
Mikacenic C, Moore R, Dmyterko V, West TE, Altemeier WA, et al. Neutrophil extracellular traps (NETs) are increased in the alveolar spaces of patients with ventilator-associated pneumonia. Critical Care. 2018; 22 (1):358. DOI: 10.1186/s13054-018-2290-8 - 99.
Nicolai A, Leunig S, Brambs S, et al. Immunothrombotic dysregulation in COVID-19 pneumonia is associated with respiratory failure and coagulopathy. Circulation. 2020; 142 (12):1176-1189 - 100.
Skendros P, Mitsios A, Chrysanthopoulou A, Mastellos DC, Metallidis S, et al. Complement and tissue factor-enriched neutrophil extracellular traps are key drivers in COVID-19 immunothrombosis. The Journal of Clinical Investigation. 2020; 130 (11):6151-6157. DOI: 10.1172/JCI141374 - 101.
Middleton EA, He XY, Robert FD, Campbell A, David NG, et al. Neutrophil extracellular traps contribute to immunothrombosis in COVID-19 acute respiratory distress syndrome. Blood. 2020; 136 (10):1169-1179 - 102.
Veras FP, Pontelli M, Silva C, Toller-Kewahisa JE, de Lima M, et al. SARS-CoV-2–triggered neutrophil extracellular traps mediate COVID-19 pathology. The Journal of Experimental Medicine. 2020; 217 (12):e20201129 - 103.
Zuo Y, Yalavarthi S, Shi H, Gockman K, Zuo M, Madison JA, et al. Neutrophil extracellular traps in COVID-19. JCI Insight. 2020; 5 (11):e138999 - 104.
Cicco S, Cicco G, Racanelli V, Vacca A. Neutrophil extracellular traps (NETs) and damage-associated molecular patterns (DAMPs): Two potential targets for COVID-19 treatment. Mediators of Inflammation. 2020; 2020 (7527953):25. DOI: 10.1155/2020/7527953 - 105.
Hu JJ, Liu X, Xia S, et al. FDA-approved disulfiram inhibits pyroptosis by blocking gasdermin D pore formation. Nature Immunology. 2020; 21 (7):736-745 - 106.
Yost CC, Schwertz H, Cody MJ, et al. Neonatal NETinhibitory factor and related peptides inhibit neutrophil extracellular trap formation. The Journal of Clinical Investigation. 2016; 126 (10):3783-3798 - 107.
Tagami T, Tosa R, Omura M, Fukushima H, Kaneko T, et al. Effect of a selective neutrophil elastase inhibitor on mortality and ventilator-free days in patients with increased extravascular lung water: A post hoc analysis of the PiCCO pulmonary edema study. Journal of Intensive Care. 2014; 2 (1):67. DOI: 10.1186/s40560-014-0067-y - 108.
Darif D, Hammi I, Kihel A, El Idrissi Saik I, Guessous F, Akarid K. The pro-inflammatory cytokines in COVID-19 pathogenesis: What goes wrong? Microbial Pathogenesis. 2021; 153 :104799 - 109.
Leng Z, Zhu R, Hou W, Feng Y, Yang Y, Han Q, et al. Transplantation of ACE2- mesenchymal stem cells improves the outcome of patients with COVID-19 pneumonia. Aging and Disease. 2020; 11 :216-228