Comparison of aptamer and antibodies.
Abstract
Early diagnosis improves the prognosis for cancer patients by allowing early intervention to slow or prevent cancer development and lethality. Aptamers are short single-stranded oligonucleotides that have a length of about 25–80 bases. They are produced chemically and extracted using the systematic evolution of ligands by exponential enrichment (SELEX). The use of aptamers as diagnostic tools in cancer is highly recommended due to their ability to recognize various cancer-related molecules and biomarkers with high affinity and specificity. Despite the clear advantages of aptamers, the potential of aptamers in cancer diagnosis is yet to be reached. This chapter will present the best available knowledge on using aptamers as the biorecognition element in the development of cancer biosensors. We will first present the advantages of aptamers in cancer diagnosis as well as various types of SELEX methods with emphasis on clinically relevant samples such as serum, whole cells, and tissue slices. We will also cover the various aptamer detection platforms, such as colorimetric, fluorescence, and electrochemical platforms. Furthermore, the updates on aptamers specific to KRAS mutation detection in cancer will be reviewed. Finally, the future direction of aptamers in cancer diagnosis will also be discussed.
Keywords
- aptamer
- SELEX
- KRAS
- cancer
- colorectal
1. Introduction
Cancer is a noncommunicable disease that is the second leading cause of death worldwide [1]. Early detection and effective treatment can save life. This is because early detection of cancer is crucial as it allows for timely intervention and treatment before cancer has a chance to metastasize to other parts of the body [2]. Furthermore, it also increased the chances of successful treatment, as the types or mutations of tumors can be screened to determine the most appropriate anticancer drugs for treatment.
Biosensor is a detection device that consists of a biorecognition element for the selective binding of target analytes and a transducer element for converting the binding event to electrical or optical signals (Figure 1) [3]. The biorecognition element typically uses biological molecules, such as enzymes, antibodies, or DNA/RNA, for rapid detection of chemicals or biomarkers in various fields such as cancer diagnostic. One of the biorecognition elements is aptamers, which are gaining more attention as they can be used to replace antibodies for biomarker discovery and specific diagnostics.
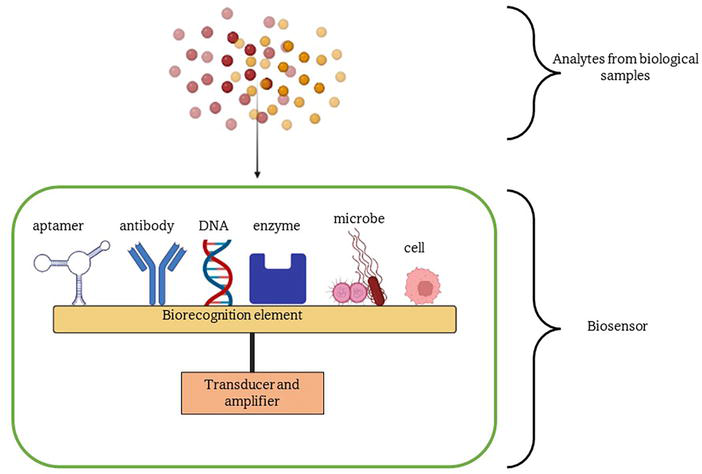
Figure 1.
Biosensor consists of two important parts, which are the biorecognition element and the transducers.
Literally, aptamer means “to fit” (aptus) in Latin. It reflects the important properties of aptamer, which are ability to fold into complex tertiary structures and ability to bind with high affinity [4]. Aptamers are typically single-stranded, 60–100 base pair of nucleic acids has gained tremendous interest as the biorecognition element in the diagnostic tool. There are DNA, RNA, XNA, and peptide aptamers that can be used to detect a wide range of targets. These aptamers differ by the selection process, affinity, and secondary structure formation. DNA aptamer was more preferred because it is more stable due to the C-H bond in the 2′ position of the deoxyribose sugar of the DNA nucleotide [5]. On the other hand, RNA aptamers need stable chemical modification for
The main diagnostic figures of merit of aptamer-based approaches are their high sensitivity, specificity, and selectivity. In cancer, the biomarkers are often present at very low concentrations in a highly complex matrix of blood or serum, which can make the diagnosis difficult [8]. Aptamers can overcome the problems as they are able to detect the presence of a target molecule at low concentrations with high accuracy. For example, high levels of cancer biomarker proteins such as prostate-specific antigen (PSA) in blood could indicate prostate cancer. An aptamer-based biosensor developed by Shayesteh et al. was able to detect PSA level as low as 20 pg./mL in human blood serum [9]. Other than that, Xia et al. developed an aptasensor to detect the presence of cancer exosomes with detection limit of 5.2×105 particles/mL [10].
The speed and convenience of aptamer-based diagnostic methods are also important figures of merit. Their rapid and convenient methods can be easily performed in a clinical setting with minimal sample preparation and processing, which are highly desirable for practical diagnostic applications. Many studies have been done to developed aptasensors that can be used for diagnostic assay in clinical diagnostic laboratories especially those that utilize optical such as colorimetric or fluorescence, and electrochemical sensing platforms [11, 12, 13, 14]. Other than that, aptamers are more stable to thermal denaturation when compared to antibodies. It also possesses a longer shelf-life and has no strict requirement for delivery and storage [11].
As summarized in Table 1, aptamers can perform beyond what antibodies can offer in diagnostic field. However, the application of aptamer in diagnostic field is still not widely used. This is due to the equipment and processes being uncommon for a clinical laboratory and lack of standardized protocols [15]. Thus, it is important to encourage aptamer-based diagnostic methods that are simple yet rapid and reliable that could be adapted in the point-of-care format for real field applications. This chapter aims to present the best available knowledge on using aptamers as the biorecognition element in the development of cancer biosensors. Different type of SELEX methods used to generate aptamers that target cancer biomarkers in clinically relevant samples such as blood serum, whole cells, and tissue slices was covered. Furthermore, the updates on aptamers specific to KRAS mutation detection in cancer are reviewed.
Aptamer | Antibodies |
---|---|
Small (~5–25 kDa) | Relatively big (>125 kDa) |
Chemically synthesis | Requires biological systems |
Low batch to batch variations | Antibodies activity varies from batch to batch |
Low immunogenicity | High immunogenicity |
Pharmacokinetics parameters can be changed on demand | Difficult to modify pharmacokinetics parameters |
Wide range of targets | Immunogenic molecules |
Efficient cellular internalization | Poor internalization into cells |
Unlimited shelf life | Limited shelf life |
Wide variety of chemical modifications to molecule for diverse functions | Limited modifications to molecule |
Sensitive to nuclease degradation | Resistant to nuclease degradation |
Very stable | Sensitive to temperature and pH changes |
Table 1.
2. SELEX methodology
Nucleic acid aptamers are derived from the systematic evolution of ligands by exponential enrichment (SELEX) methodology. In this method, an aptamer that has 60–100 nucleotides that can bind specifically to target is isolated from a random library of double-stranded DNA (dsDNA). Then, the dsDNA library is used to synthesize a single-stranded DNA (ssDNA) library or undergoes
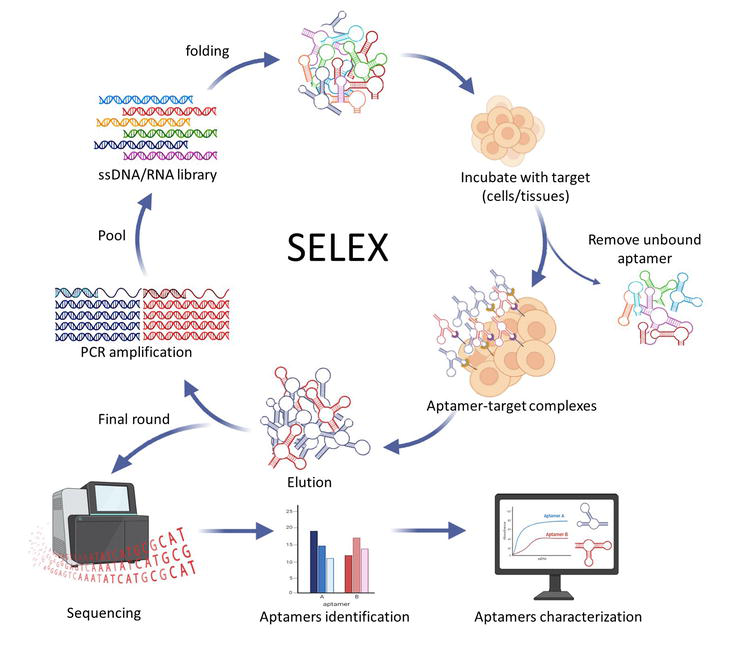
Figure 2.
SELEX process.
A variety of SELEX has been developed to obtain aptamers for various targets for cancer diagnosis and treatment (Table 2). To name a few, there are cell-SELEX, tissue-SELEX, SOMAmer-SELEX,
Type of cancer | Aptamer | Type | SELEX | Biomarkers | Ref. |
---|---|---|---|---|---|
Bladder | EN2-binding ssDNA aptamer spl3 | DNA DNA | N/A Cell-SELEX | Engrailed-2(EN-2) Cytoskeleton-associated protein 4 (CKAP4) | [18] [19] |
Colorectal | Apta-3, Apta-5 Seq-2 ApC1 | DNA DNA DNA | Protein-SELEX Serum-SELEX Cell-SELEX | Carcinoembryonic antigen (CEA) in T84 cells. N/A Caco-2 | [20] [21] [22] |
Prostate | A9, A9g, A10, A10-3, A10L, A10-3.2, A10-3-J | RNA | Cell-SELEX | PSMA | [23, 24, 25, 26] |
Wy-5a, DML-7, AMH, CSC1, CSC13, E3, PSap4#5, S3.1/S2.2 | DNA DNA | Cell-SELEX Cell-SELEX | Prostate cancer cell lines, MUC-1 protein | [27, 28, 29, 30, 31, 32] [33] | |
Gastric | Seq-3, Seq-6, Seq-19, Seq-54 dual-aptamer (EpCAM and PTK7)-modified immunomagnetic Fe3O4 particles (IMNs) NCL-Apt-miRNA let-7d chimera PDGC21-T, PDGC21-T-QD cy-apt 20 | DNA DNA DNA DNA DNA | Serum-SELEX Cell-SELEX N/A Cell-SELEX Cell-SELEX | APOA1, APOA4, PARD2, Importin subunit α-1 Circulating tumor cells (CTCs) Nucleolin BGC-823 cells, PDGC tissues AGS cells | [34] [35] [36] [37] [38] |
Breast | SE15-8 ex-50.T | RNA RNA | Exo-SELEX | ErbB2 protein Exosomes | [39] [40] |
CD63-1 and CD63-2 sk6Ea MF3Ec SKBR3-R1Tr Apt1, Apt2 | DNA DNA DNA DNA DNA | Competitive-SELEX Cell-SELEX Cell-SELEX 3D cell-SELEX Cell-SELEX Cell-SELEX | CD63 protein HER2 protein Cell membrane protein PHB2, Luminal A breast cancer subtype SKBR3 cells MDA-MB-231 cells | [41] [42] [43], [44] [45] [46] | |
Cervical | HPV-07 C-9S | DNA DNA | SOMAmer-SELEX Cell-SELEX | Type 16 virus-like-particle (VLP) Ca Ski cells, Hela cells | [47] [48] |
Hepatocellular | LY-1, LY-13, LY-46, LY-32, LY-27/45, LY-7/43 | DNA | Cell SELEX | HCCLM9 cells | [49] |
Various | Ep-DT3-DY647 AS1411 | RNA DNA | Cancer stem cells (CTCs) Nucleolin | [50] [51, 52, 53, 54] |
Table 2.
Aptamers for cancer.
2.1 Cell-SELEX
In general, cell-SELEX uses intact and living cells as the target to screen a panel of oligonucleotides against multiple cell surface proteins and other macromolecules [55]. The target cells usually were cancer cells, normal cells as contrast cells, or genetically engineered cells that expressed target proteins. This type of SELEX is effective in developing aptamers for cancer biomarker discovery. A major advantage of using cell-SELEX is that the aptamer selection can be performed without prior knowledge of the target protein [56]. Furthermore, aptamers generated using this process can recognize their target in native form as it is not affected by protein purification processes [56]. However, it is important to avoid cell death or remove dead cells population during the selection process. This is because dead cells can cause false positives due to nonspecific uptake and binding of oligonucleotides, thus hampered the selection process [56, 57]. High-speed fluorescence activating sorting (FACS) can be used to separate between live and dead or damaged cells [58]. Aptamer selection using this type of SELEX is costly and challenging due to the dynamic and multifaceted structure of the cell membrane as it contains various interacting molecules, such as phospholipids, receptors, transporters, and membrane proteins. Membrane proteins are often embedded within or associated with cell membranes, thus separating aptamers that bind to them can be difficult.
In cell-SELEX method, whole cells are used as targets for aptamers selection. The aptamers selected using this type of SELEX were able to recognize cell surface markers or other cell-associated targets [59]. There were various types of aptamers selected using cell-SELEX that showed the prospects as diagnostics tool in cancer. It has been shown that nucleolin, a cell-surface protein, was often over-expressed in multiple cancer cells [60, 61, 62]. Studies showed that AS1411 which is an aptamer selected using cell-SELEX can bind to nucleolin with high affinity [52, 63]. AS1411 was used together with other conjugates for targeted cancer cell imaging. For example, AS1411 aptamer labeled with positron emission tomography (PET) was used as diagnostic imaging agent to detect lung cancer [54]. It can also be conjugated with proteolysis targeting chimeras (PROTAC), gold nanoclusters (GNCs), or carbon dots (CDs) for use in cancer cells targeted imaging [51, 53, 64, 65]. Another study demonstrated that utilizing this aptamer linked with a polymer probe can enable precise and high resolution of nucleolin on the cell surface with selectivity [66].
Prostate-specific membrane antigen (PSMA) is a transmembrane protein, expressed by prostate cancer, hence excellent target for prostate cancer screening [67]. PSMA-specific RNA aptamers, A10 and A9, and DNA PSA-specific aptamer PSap4#5 were the most studied aptamer for prostate cancer diagnosis [68]. A modified version of the A10 aptamer sequence with 2′-fluoro pyrimidine modification that is more stable and less prone to degradation in biological fluid has been developed to make it a potentially more effective diagnostic tool for prostate cancer.
2.2 Tissue-SELEX
Aptamers selected using the tissue-SELEX method could improve target recognition
2.3 Protein-SELEX
In protein-SELEX, a library of random nucleic acid sequences is incubated with the target protein of interest. The sequences that bind to the protein are then separated from the unbound sequences by using chromatography. In comparison with conventional SELEX, this type of SELEX is utilized for the discovery of novel aptamer for known purified proteins. However, aptamers selected using protein-SELEX may not be able to recognize their corresponding targets
2.4 Serum-SELEX
Serum is a complex mixture of proteins, lipids, hormones, enzymes, electrolytes, and other small molecules. These components can interfere with the detection of cancer biomarkers. Interestingly, aptamers have very high specificity as they can bind to specific epitope on the target molecule, thus lower false-positive rate compared to antibodies, which sometimes can cross-react with similar molecules. In serum-SELEX, the pooled serum is used as target to ensure the diversity of specific target [72]. Negative selection or also known as counter-selection is the initial step that is designed to eliminate sequences that bind to normal serum (Figure 2). After exposure to normal serum, the remaining unbound sequences are then carried forward to the next round for positive selection [34]. The unbound sequences are exposed to the target molecule again for further enrichment. Thus, aptamers with improved specificity and selectivity can be obtained for diagnostic application. For example, Apt-5 can bind to human CLEC3b, which plays an important role in infection, inflammation, and tumor immunity [73, 74]. Aptamer Apt-5 can be used as a molecular probe to detect lung cancer. Other than that, a study by Li et al. successfully identified six different aptamers that have high specificity and strong affinity to the sera of lung cancer patients [75]. These aptamers could be further developed as probes for early diagnosis of lung cancer (Figure 3).
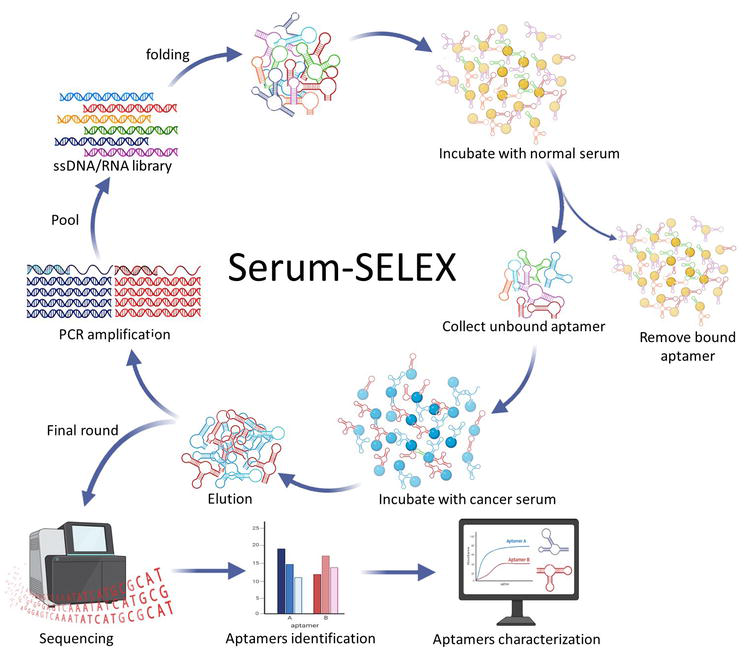
Figure 3.
Serum SELEX.
2.5 Toggle-SELEX
Toggle-SELEX is used to select a species of the cross-reactive aptamer. This type of SELEX is used to generate aptamers that can bind to two different targets. During the selection process, the target molecules are alternated in each round; thus, the selected aptamers can bind both targets most likely to domains conserved between the protein targets. One of the aptamers generated using this type of SELEX was Toggle-25 thrombin aptamer that is used for plasma clot formation and platelet activation [76]. Aptamers generated from toggle-SELEX will facilitate the transition of aptamers from animal models to human subjects.
3. Aptamer for rapid testing and detection platforms
Early detection of cancer often requires the testing of bodily fluids, such as blood, serum or plasma, and tissues. Most of the current design of aptamer-based detection systems often does not account for interfering substances in biological fluids. Using biological fluids such as blood, urine or serum for cancer diagnostic has several challenges. The main reason is a low abundance of cancer biomarkers in blood, making it difficult to detect them using conventional diagnostic assay. This requires the development of highly sensitive and specific assays to accurately detect and quantify cancer biomarkers in blood.
Blood has high nuclease activity; thus, the aptamers must be modified to increase their stability, such as adding sugar, base, or backbone modifications. In addition, the serum is also used for cancer biomarkers detection. However, the components of blood and serum are complex and often difficult to detect low-concentration cancer biomarkers, which hinder early cancer diagnosis. One way to increase the stability and resistance of aptamers against 3′-exonucleases in human serum is by capping the 3′-end with inverted deoxy-thymidine or adding 3′-biotin modification [77]. Studies show that 3′ biotin aptamer modification results in enhanced 3′-nucleases resistance and decrease aptamer clearance from blood circulation
The majority of aptamer-based analytical systems (aptasensors) use optical-based test system that includes colorimetric, fluorescent, and luminescence. Colorimetric aptasensors, such as aptamer-linked immunosorbent assay (ALISA), enzyme-linked oligonucleotide assay (ELONA), or enzyme-linked aptamer assay (ELASA), are dependent on color change of the solution by naked eyes or quantified by using spectrophotometer [80]. Aptamer-based ELISA uses aptamers instead of antibodies to detect the presence of a specific target in a sample. The aptamers can be immobilized on a plate and capture the target, which will be detected using a secondary reagent. Sandwich-type colorimetric aptasensors are known for their high specificity and selectivity, but they typically require the use of aptamer/antibody pairs. Thus, developing completely antibody-free or aptamer/aptamer sandwich-type assays would improve the sensitivity and specificity [11] Other than that, an aptamer can also be immobilized on a test strip and will bind to a specific target such as cancer-related protein in aptamer-based lateral flow assay. This low-cost method has been used to detect various cancer biomarkers such as CD63, CA125, and human osteopontin utilizing nanogold particles as the visualization probe [81, 82, 83]. Other than that, the combination of surface-enhanced Raman spectroscopy and lateral flow assay (SERS-LFA) was able to produce convenient, rapid, and sensitive detection of thrombin and platelet-derived growth factor-BB (PDGF-BB) associated with the prostate cancer [84]. Man et al. have developed a chemiluminescence enzyme-linked aptamer assay to simultaneously detect VEGF and CEA cancer biomarkers [85].
Fluorescence-based assays can be used to detect the binding of aptamer to its target molecule by measuring changes in fluorescence polarization or intensity. Labeling the aptamer or target molecules using fluorophores and their signal detection is based on fluorescence resonance energy transfer (FRET), which will reflect the extent of the aptamer-target binding process, thus allowing the quantitative measurement of the target concentration [86]. Several fluorescence biosensors based on aptamers have demonstrated promising results in biological samples such as serum, blood, and urine. Study by Liang et al. showed that aptamers labeled using fluorescence dye and graphene oxide can be used to detect oncoprotein PDGF-BB [87]. In another study, fluorescent probes were used to detect angiogenin in serum samples of healthy and cancer patients [88, 89]. Overall, fluorescence-based assays for aptamer detection can offer real-time monitoring, multiplexing, and nondestructive detection capabilities as reviewed in Ref. [86].
Electrochemical detection platform is based on the measurement of electrical properties such as current or voltage changes due to the binding of an aptamer to its target. The electrode surfaces used in electrochemical aptamer detection include gold electrode [90], carbon electrode [91, 92], and screen-printed electrode [93]. These platforms can support point-of-care testing (POCT) as it allows real-time detection of cancer biomarkers and enables faster diagnosis and treatment [94].
4. Aptamers specific to KRAS mutation
RAS, a guanine nucleotide-binding protein, functions as a transmission of extracellular signals to the interior of the cell. The RAS family is mainly involved in the Ras-Raf-MAPK cell signaling pathway, which plays a key role in cell proliferation, differentiation, and survival [95]. RAS exists in two conformations that can be reversibly switched to an active (guanosine triphosphate [GTP]) or inactive (guanosine diphosphate [GDP]) state through interaction with guanine nucleotide exchange factors or GTPase-activating proteins (GAP). Mutation in RAS causes defective intrinsic GTPase function leading to persistent activations of Ras-Raf-MAPK pathway. Therefore, RAS superfamily is known as proto-oncogenes, involving Harvey-RAS (HRAS), neuroblastoma-RAS (NRAS), and two splicing variants of Kristen-RAS (KRAS) (KRAS4A and KRAS4B) [96]. About 20% of human tumors are associated with point mutations in the GTPase domain of the RAS gene. Oncogenic NRAS mutations are found in 15–30% of cutaneous malignant melanoma, whereas HRAS mutations are found mostly in oral cavity tumors and hepatocellular carcinomas [97]. To date, oncogenic KRAS mutations are the most frequent and are commonly found in pancreatic (>80%), colorectal (40–50%), and lung (30–50%) carcinomas [98]. Overall, mutations in KRAS genes are necessary for an early event and maintenance of tumorigenesis.
Substitution mutations in the KRAS gene account for 60% of all colorectal cancer cases. Of those, approximately 83% involve codon 12 and 14% involve codon 13, while codon 61 accounts for a minor proportion (2%) [99]. These allelic mutations are located near the GTP binding site, whereby the most clinically frequent substitution from glycine (G) to aspartate (D) was found to occur on codon 12. In primary metastatic colorectal carcinoma, a change in amino acids from glycine (G) to valine (V) at codon 12 occurred more often and this was linked to early mortality. Li et al. suggested that G12D and G12V mutations were independent prognostic factors for poor prognosis in colorectal cancer patients [100]. Similarly, the most frequent substitution of glycine (G) with aspartate (D) in codon 13 has been shown to be associated with the reduced survival rates in colorectal cancer patients [101].
Although RAS isoforms are highly conserved proteins, their carboxy-terminal domain is diverse in terms of structure and posttranslational modifications. After examining the lysine-rich polybasic carboxy-terminal region of KRAS protein, Tanaka and his group were able to identify RNA aptamers that directly target the KRAS4B isotype protein [102]. They applied an improved SELEX method using isothermal RNA amplification to isolated RNA aptamers targeting activated KRAS proteins. A farnesylated peptide model was used to mimic the carboxy-terminal region of KRAS protein for the selection of RNA aptamers against an affinity column. After eluting out the specific binding RNA aptamers from the column, sequences of two binding aptamers were revealed. The binding affinity of these aptamers to the farnesylated peptide is 10-fold stronger than binding to nonfarnesylated peptide indicating these aptamers could recognize the hydrophobic farnesyl moiety of KRAS4B.
In contrast to the discovery of aptamers targeting KRAS4B, C-terminal domain-binding aptamers were unable to distinguish the oncogenic mutant KRAS protein from wild-type KRAS protein. Hence, an RNA aptamer that specifically binds to mutant KRAS protein was engineered using SELEX method [103]. The aptamer selected in this study has a 50-fold greater affinity for mutant KRAS G12V than for wild-type KRAS, whereby KRAS G12V are known to account for 33.6% of total KRAS mutations. In order to study the targets, cDNA-encoding human wild-type KRAS and KRAS G12V proteins were cloned into pET28a(+) expression vector and expressed in
5. Conclusion and future direction
Cancer diagnosis often relies on detecting specific biomarkers such as cancer antigens or circulating tumor cells in the serum, cancer tissues, or blood. Aptamers can be designed to bind to these biomarkers and used as the basis for diagnostic tests. They also hold promise as a powerful tool for cancer diagnosis due to their specificity, sensitivity, and versatility. Most cancer biomarkers discovered today are shared among two or more cancer types. Thus, multiplexing of aptamer-based diagnostic assays could greatly improve the accuracy and sensitivity of cancer diagnosis. For colorectal cancer, the search for KRAS mutation variants aptamers is of utmost importance to distinguish patients who will respond to therapy such as epidermal growth factor-based therapy. Incorporation of the existing biosensor device (e.g., glucometer) with the aptamer-based assay could enhance the translation of aptamer-based assay by omitting the design manufacturing and validation of the prototype [80]. Overall, more research is needed to develop clinically relevant aptamers as a diagnostic tool that holds great promise for the future.
Acknowledgments
We would like to thank UCSI University for funding through the Research Excellence and Innovation Grant (REIG-FAS-2022/047).
References
- 1.
Sung H et al. Global cancer statistics 2020: GLOBOCAN estimates of incidence and mortality worldwide for 36 cancers in 185 countries. CA: A Cancer Journal for Clinicians. 2021; 71 (3):209-249 - 2.
Ginsburg O et al. Breast cancer early detection: A phased approach to implementation. Cancer. 2020; 126 (S10):2379-2393 - 3.
Kawamura A, Miyata T. Biosensors. In: Ebara M, editor. Biomaterials Nanoarchitectonics. New York: Elsevier; 2016. pp. 157-176 - 4.
Thiel KW, Giangrande PH. Therapeutic applications of DNA and RNA aptamers. Oligonucleotides. 2009; 19 (3):209-222 - 5.
Kim DH, Seo JM, Shin KJ, Yang SG. Design and clinical developments of aptamer-drug conjugates for targeted cancer therapy. Biomaterials Research. 2021; 25 (1):42 - 6.
Odeh F et al. Aptamers chemistry: Chemical modifications and conjugation strategies. Molecules. 2020; 25 (1):3 - 7.
Kohlberger M, Gadermaier G. SELEX: Critical factors and optimization strategies for successful aptamer selection. Biotechnology and Applied Biochemistry. 2022; 69 (5):1771-1792 - 8.
Kumar Kulabhusan P, Hussain B, Yüce M. Current perspectives on aptamers as diagnostic tools and therapeutic agents. Pharmaceutics. 2020; 12 (7):646 - 9.
Shayesteh OH, Ghavami R. A novel label-free colorimetric aptasensor for sensitive determination of PSA biomarker using gold nanoparticles and a cationic polymer in human serum. Spectrochimica Acta. Part A, Molecular and Biomolecular Spectroscopy. 2020; 226 :117644 - 10.
Xia Y et al. A dual-modal aptasensor based on a multifunctional acridone derivate for exosomes detection. Analytica Chimica Acta. 2022; 1191 :339279 - 11.
Davydova A, Vorobyeva M. Aptamer-based biosensors for the colorimetric detection of blood biomarkers: Paving the way to clinical laboratory testing. Biomedicine. 2022; 10 (7):1606 - 12.
Wilkirson EC et al. Affinity-based electrochemical sensors for biomolecular detection in whole blood. Analytical and Bioanalytical Chemistry. 2023; 14 :1-20 - 13.
Labib M, Sargent EH, Kelley SO. Electrochemical methods for the analysis of clinically relevant biomolecules. Chemical Reviews. 2016; 116 (16):9001-9090 - 14.
Wang H, Wu T, Li M, Tao Y. Recent advances in nanomaterials for colorimetric cancer detection. Journal of Materials Chemistry B. 2021; 9 (4):921-938 - 15.
Lakhin AV, Tarantul VZ, Gening LV. Aptamers: Problems, solutions and prospects. Acta Naturae. 2013; 5 (4):34-43 - 16.
Rahimizadeh K et al. Development of cell-specific aptamers: Recent advances and insight into the selection procedures. Molecules. 2017; 22 (12):2070 - 17.
Komarova N, Kuznetsov A. Inside the black box: What makes SELEX better? Molecules. 2019; 24 (19):3598 - 18.
Kim E, Kang M, Ban C. Aptamer-antibody hybrid ELONA that uses hybridization chain reaction to detect a urinary biomarker EN2 for bladder and prostate cancer. Scientific Reports. 2022; 12 (1):11523 - 19.
Sun X et al. Elucidation of CKAP4-remodeled cell mechanics in driving metastasis of bladder cancer through aptamer-based target discovery. Proceedings of the National Academy of Sciences of The United States of America. 2022; 119 (16):e2110500119 - 20.
Izabella Abreu, de Melo M, Rodrigues Correa C, da Silva Cunha P, Miranda, de Góes A, Assis Gomes D, Silva, Ribeiro, de Andrade A. DNA aptamers selection for carcinoembryonic antigen (CEA). Bioorganic & Medicinal Chemistry Letters. 2020; 30 (15):127278 - 21.
Li K et al. Selection and preliminary application of a single stranded DNA aptamer targeting colorectal cancer serum. RSC Advances. 2019; 9 (66):38867-38876 - 22.
Maimaitiyiming Y, Yang C, Wang Y, Hussain L, Naranmandura H. Selection and characterization of novel DNA aptamer against colorectal carcinoma Caco-2 cells. Biotechnology and Applied Biochemistry. 2019; 66 (3):412-418 - 23.
Lupold SE. Aptamers and apple pies: A mini-review of PSMA aptamers and lessons from Donald S. Coffey. American Journal of Clinical and Experimental Urology. 2018; 6 (2):78-86 - 24.
Leach J, Wang A, Ye K, Jin S. A RNA-DNA hybrid aptamer for nanoparticle-based prostate tumor targeted drug delivery. International Journal of Molecular Sciences. 2016; 17 (3):380 - 25.
Dassie JP et al. Systemic administration of optimized aptamer-siRNA chimeras promotes regression of PSMA-expressing tumors. Nature Biotechnology. 2009; 27 (9):839-846 - 26.
Rockey WM et al. Rational truncation of an RNA aptamer to prostate-specific membrane antigen using computational structural Modeling. Nucleic Acid Therapeutics. 2011; 21 (5):299-314 - 27.
Wang Y et al. DNA aptamer evolved by cell-SELEX for recognition of prostate cancer. PLoS One. 2014; 9 (6):e100243 - 28.
Duan M et al. Selection and characterization of DNA aptamer for metastatic prostate cancer recognition and tissue imaging. Oncotarget. 2016; 7 (24):36436-36446 - 29.
Kouhpayeh S et al. Antiproliferative effect of a synthetic aptamer mimicking androgen response elements in the LNCaP cell line. Cancer Gene Therapy. 2016; 23 (8):254-257 - 30.
Wang J et al. Aptamer-conjugated Nanorods for targeted Photothermal therapy of prostate cancer stem cells. Chemistry, an Asian Journal. 2013; 8 (10):2417-2422 - 31.
Powell Gray B et al. Tunable cytotoxic aptamer–drug conjugates for the treatment of prostate cancer. Proceedings of the National Academy of Sciences. 2018; 115 (18):4761-4766 - 32.
Savory N, Abe K, Sode K, Ikebukuro K. Selection of DNA aptamer against prostate specific antigen using a genetic algorithm and application to sensing. Biosensors & Bioelectronics. 2010; 26 (4):1386-1391 - 33.
Crulhas BP, Karpik AE, Delella FK, Castro GR, Pedrosa VA. Electrochemical aptamer-based biosensor developed to monitor PSA and VEGF released by prostate cancer cells. Analytical and Bioanalytical Chemistry. 2017; 409 (29):6771-6780 - 34.
Zheng Y et al. DNA aptamers from whole-serum SELEX as new diagnostic agents against gastric cancer. RSC Advances. 2019; 9 (2):950-957 - 35.
Li C et al. Dual-aptamer-targeted Immunomagnetic nanoparticles to accurately explore the correlations between circulating tumor cells and gastric cancer. ACS Applied Materials & Interfaces. 2022; 14 (6):7646-7658 - 36.
Ramezanpour M, Daei P, Tabarzad M, Khanaki K, Elmi A, Barati M. Preliminary study on the effect of nucleolin specific aptamer–miRNA let-7d chimera on Janus kinase-2 expression level and activity in gastric cancer (MKN-45) cells. Molecular Biology Reports. 2019; 46 (1):207-215 - 37.
Li W, Wang S, Zhou L, Cheng Y, Fang J. An ssDNA aptamer selected by cell-SELEX for the targeted imaging of poorly differentiated gastric cancer tissue. Talanta. 2019; 199 :634-642 - 38.
Cao HY, Yuan AH, Chen W, Shi XS, Miao Y. A DNA aptamer with high affinity and specificity for molecular recognition and targeting therapy of gastric cancer. BMC Cancer. 2014; 14 (1):699 - 39.
Kim MY, Jeong S. In vitro selection of RNA aptamer and specific targeting of ErbB2 in breast cancer cells. Nucleic Acid Therapeutics. 2011; 21 (3):173-178 - 40.
Esposito CL et al. Identification of a novel RNA aptamer that selectively targets breast cancer exosomes. Molecular Therapy - Nucleic Acids. 2021; 23 :982-994 - 41.
Song Z, Mao J, Barrero R, Wang P, Zhang F, Wang T. Development of a CD63 aptamer for efficient cancer immunochemistry and immunoaffinity-based exosome isolation. Molecules. 2020; 25 (23):5585 - 42.
Liu M et al. An aptamer-based probe for molecular subtyping of breast cancer. Theranostics. 2018; 8 (20):5772-5783 - 43.
Liu M, Wang Z, Li S, Deng Y, He N. Identification of PHB2 as a potential biomarker of luminal a breast cancer cells using a cell-specific aptamer. ACS Applied Materials & Interfaces. 2022; 14 (46):51593-51601 - 44.
Liu M et al. Precise discrimination of luminal a breast cancer subtype using an aptamer in vitro and in vivo. Nanoscale. 2020; 12 (38):19689-19701 - 45.
Nelissen FHT, Peeters WJM, Roelofs TP, Nagelkerke A, Span PN, Heus HA. Improving breast cancer treatment specificity using aptamers obtained by 3D cell-SELEX. Pharmaceuticals. 2021; 14 (4):349 - 46.
Ferreira D et al. Selection of aptamers against triple negative breast cancer cells using high throughput sequencing. Scientific Reports. 2021; 11 (1):8614 - 47.
Trausch JJ, Shank-Retzlaff M, Verch T. Development and characterization of an HPV Type-16 specific modified DNA aptamer for the improvement of potency assays. Analytical Chemistry. 2017; 89 (6):3554-3561 - 48.
Wang J et al. In vitro selection of a DNA aptamer by cell-SELEX as a molecular probe for cervical cancer recognition and imaging. Journal of Molecular Evolution. 2019; 87 (2-3):72-82 - 49.
Chen H et al. Subtractive cell-SELEX selection of DNA aptamers binding specifically and selectively to hepatocellular carcinoma cells with high metastatic potential. BioMed Research International. 2016; 2016 :5735869 - 50.
Shigdar S, Lin J, Yu Y, Pastuovic M, Wei M, Duan W. RNA aptamer against a cancer stem cell marker epithelial cell adhesion molecule. Cancer Science. 2011; 102 (5):991-998 - 51.
Kong T, Zhou R, Zhang Y, Hao L, Cai X, Zhu B. AS1411 aptamer modified carbon dots via polyethylenimine-assisted strategy for efficient targeted cancer cell imaging. Cell Proliferation. 2020; 53 (1):e12713 - 52.
Jing Y, Cai M, Zhou L, Jiang J, Gao J, Wang H. Aptamer AS1411 utilized for super-resolution imaging of nucleolin. Talanta. 2020; 217 :121037 - 53.
He S, Gao F, Ma J, Ma H, Dong G, Sheng C. Aptamer-PROTAC conjugates (APCs) for tumor-specific targeting in breast cancer. Angewandte Chemie International Edition in English. 2021; 60 (43):23299-23305 - 54.
Li J et al. Aptamer imaging with Cu-64 labeled AS1411: Preliminary assessment in lung cancer. Nuclear Medicine and Biology. 2014; 41 (2):179-185 - 55.
Sola M et al. Aptamers against live targets: Is In vivo SELEX finally coming to the edge? Molecular Therapy. Nucleic Acids. 2020; 21 :192-204 - 56.
Chen M et al. Development of cell-SELEX technology and its application in cancer diagnosis and therapy. International Journal of Molecular Sciences. 2016; 17 (12):2079 - 57.
Chen C, Zhou S, Cai Y, Tang F. Nucleic acid aptamer application in diagnosis and therapy of colorectal cancer based on cell-SELEX technology. NPJ Precision Oncology. 2017; 1 (1):37 - 58.
Germer K, Leonard M, Zhang X. RNA aptamers and their therapeutic and diagnostic applications. International Journal of Biochemistry and Molecular Biology. 2013; 4 (1):27-40 - 59.
Cibiel A, Dupont DM, Ducongé F. Methods to identify aptamers against cell surface biomarkers. Pharmaceuticals. 2011; 4 (9):1216-1235 - 60.
Wang Y et al. Nucleolin-targeted extracellular vesicles as a versatile platform for biologics delivery to breast cancer. Theranostics. 2017; 7 (5):1360-1372 - 61.
Carvalho LS, Gonçalves N, Fonseca NA, Moreira JN. Cancer stem cells and nucleolin as drivers of carcinogenesis. Pharmaceuticals. 2021; 14 (1):60 - 62.
Brignole C et al. Cell surface Nucleolin represents a novel cellular target for neuroblastoma therapy. Journal of Experimental & Clinical Cancer Research. 2021; 40 (1):180 - 63.
Vindigni G et al. AS1411 aptamer linked to DNA nanostructures diverts its traffic inside cancer cells and improves its therapeutic efficacy. Pharmaceutics. 2021; 13 (10):1671 - 64.
Ghahremani F, Shahbazi-Gahrouei D, Kefayat A, Motaghi H, Mehrgardi MA, Javanmard SH. AS1411 aptamer conjugated gold nanoclusters as a targeted radiosensitizer for megavoltage radiation therapy of 4T1 breast cancer cells. RSC Advances. 2018; 8 (8):4249-4258 - 65.
Motaghi H, Mehrgardi MA, Bouvet P. Carbon dots-AS1411 aptamer nanoconjugate for ultrasensitive spectrofluorometric detection of cancer cells. Scientific Reports. 2017; 7 (1):10513 - 66.
Fabre L et al. Fluorescent polymer-AS1411-aptamer probe for dSTORM super-resolution imaging of endogenous Nucleolin. Biomacromolecules. 2022; 23 (6):2302-2314 - 67.
Haberkorn U, Eder M, Kopka K, Babich JW, Eisenhut M. New strategies in prostate cancer: Prostate-specific membrane antigen (PSMA) ligands for diagnosis and therapy. Clinical Cancer Research. 2016; 22 (1):9-15 - 68.
Campos-Fernández E et al. The use of aptamers in prostate cancer: A systematic review of theranostic applications. Clinical Biochemistry. 2021; 93 :9-25 - 69.
Dua P, Kim S, Lee D. Nucleic acid aptamers targeting cell-surface proteins. Methods. 2011; 54 (2):215-225 - 70.
Li L et al. Identification of a new DNA aptamer by tissue-SELEX for cancer recognition and imaging. Analytical Chemistry. 2021; 93 (19):7369-7377 - 71.
Tsai Y-C, Lin C-S, Lin C-N, Hsu K-F, Lee G-B. Screening aptamers targeting the cell membranes of clinical cancer tissues on an integrated microfluidic system. Sensors and Actuators B Chemical. 2021; 330 :129334 - 72.
Wang T et al. A universal strategy designed for selecting bench-to-bedside aptamers to serum and validated in hepatocellular carcinoma diagnosis. Microchemical Journal. 2019; 150 :104152 - 73.
Guo Y et al. CLEC3B identified as a potential lung cancer biomarker in serum by aptamer-capture technology. Chemistry Select. 2021; 6 (22):5640-5645 - 74.
Zhao Y et al. Identification of a novel DNA aptamer that selectively targets lung cancer serum. RSC Advances. 2021; 11 (53):33759-33769 - 75.
Li K et al. Screening of specific nucleic acid aptamers binding tumor markers in the serum of the lung cancer patients and identification of their activities. Tumor Biology. 2017; 39 (7):101042831771712 - 76.
White R et al. Generation of species cross-reactive aptamers using ‘toggle’ SELEX. Molecular Therapy. 2001; 4 (6):567-573 - 77.
Zhuo Z et al. Recent advances in SELEX technology and aptamer applications in biomedicine. International Journal of Molecular Sciences. 2017; 18 (10):2142 - 78.
Dougan H, Lyster DM, Vo CV, Stafford A, Weitz JI, Hobbs JB. Extending the lifetime of anticoagulant oligodeoxynucleotide aptamers in blood. Nuclear Medicine and Biology. 2000; 27 (3):289-297 - 79.
Ni S et al. Recent Progress in aptamer discoveries and modifications for therapeutic applications. ACS Applied Materials & Interfaces. 2021; 13 (8):9500-9519 - 80.
Sharma TK, Bruno JG, Dhiman A. ABCs of DNA aptamer and related assay development. Biotechnology Advances. 2017; 35 (2):275-301 - 81.
Yu Q et al. Development of a lateral flow aptamer assay strip for facile identification of theranostic exosomes isolated from human lung carcinoma cells. Analytical Biochemistry. 2020; 594 :113591 - 82.
Mukama O et al. A highly sensitive and specific lateral flow aptasensor for the detection of human osteopontin. Talanta. 2020; 210 :120624 - 83.
Tripathi P, Sachan M, Nara S. Novel ssDNA ligand against ovarian cancer biomarker CA125 with promising diagnostic potential. Frontiers in Chemistry. 2020; 8 :400 - 84.
Cao X, Song Q, Sun Y, Mao Y, Lu W, Li L. A SERS-LFA biosensor combined with aptamer recognition for simultaneous detection of thrombin and PDGF-BB in prostate cancer plasma. Nanotechnology. 2021; 32 (44):445101 - 85.
Man J et al. Simultaneous detection of VEGF and CEA by time-resolved Chemiluminescence enzyme-linked aptamer assay. International Journal of Nanomedicine. 2020; 15 :9975-9985 - 86.
Musumeci D, Platella C, Riccardi C, Moccia F, Montesarchio D. Fluorescence sensing using DNA aptamers in cancer research and clinical diagnostics. Cancers (Basel). 2017; 9 (12):174 - 87.
Liang J, Wei R, He S, Liu Y, Guo L, Li L. A highly sensitive and selective aptasensor based on graphene oxide fluorescence resonance energy transfer for the rapid determination of oncoprotein PDGF-BB. Analyst. 2013; 138 (6):1726 - 88.
Li W, Yang X, Wang K, Tan W, Li H, Ma C. FRET-based aptamer probe for rapid angiogenin detection. Talanta. 2008; 75 (3):770-774 - 89.
Li W et al. Real-time imaging of protein internalization using aptamer conjugates. Analytical Chemistry. 2008; 80 (13):5002-5008. DOI: 10.1021/ac800930q - 90.
Ilyas A, Asghar W, Allen PB, Duhon H, Ellington AD, Iqbal SM. Electrical detection of cancer biomarker using aptamers with nanogap break-junctions. Nanotechnology. 2012; 23 (27):275502 - 91.
Yeh F-Y, Liu T-Y, Tseng I-H, Yang C-W, Lu L-C, Lin C-S. Gold nanoparticles conjugates-amplified aptamer immunosensing screen-printed carbon electrode strips for thrombin detection. Biosensors & Bioelectronics. 2014; 61 :336-343 - 92.
Khoshfetrat SM, Mehrgardi MA. Amplified detection of leukemia cancer cells using an aptamer-conjugated gold-coated magnetic nanoparticles on a nitrogen-doped graphene modified electrode. Bioelectrochemistry. 2017; 114 :24-32 - 93.
Ferreira DC, Batistuti MR, Bachour B, Mulato M. Aptasensor based on screen-printed electrode for breast cancer detection in undiluted human serum. Bioelectrochemistry. 2021; 137 :107586 - 94.
Omage JI et al. Cancer diagnostics and early detection using electrochemical Aptasensors. Micromachines (Basel). 2022; 13 (4):522 - 95.
Fernandez-Medarde A, Santos E. Ras in cancer and developmental diseases. Genes & Cancer. 2011; 2 (3):344-358 - 96.
Burge RA, Hobbs GA. Not all RAS mutations are equal: A detailed review of the functional diversity of RAS hot spot mutations. Advances in Cancer Research. 2022; 153 :29-61 - 97.
Kodaz H et al. Frequency of RAS mutations (KRAS, NRAS, HRAS) in human solid cancer. Eurasian Journal of Medicine and Oncology. 2017; 1 (1):1-7 - 98.
Timar J, Kashofer K. Molecular epidemiology and diagnostics of KRAS mutations in human cancer. Cancer and Metastasis Reviews. 2020; 39 (4):1029-1038 - 99.
Hobbs GA, Der CJ, Rossman KL. RAS isoforms and mutations in cancer at a glance. Journal of Cell Science. 2016; 129 (7):1287-1292 - 100.
Li W et al. Not all mutations of KRAS predict poor prognosis in patients with colorectal cancer. International Journal of Clinical and Experimental Pathology. 2019; 12 (3):957-967 - 101.
Badic B et al. Predictors of survival in elderly patients with metastatic colon cancer: A population-based cohort study. Cancers (Basel). 2022; 14 (21):5208 - 102.
Tanaka Y, Akagi K, Nakamura Y, Kozu T. RNA aptamers targeting the carboxyl terminus of KRAS Oncoprotein generated by an improved SELEX with isothermal RNA amplification. Oligonucleotides. 2007; 17 (1):12-21 - 103.
Jeong S, Han SR, Lee YJ, Kim JH, Lee S-W. Identification of RNA aptamer specific to mutant KRAS protein. Oligonucleotides. 2010; 20 (3):155-161