Experimental parameters for smooth surfaces,
Abstract
This chapter discusses different measurement techniques of a low-speed wind tunnel designed and built at the Faculty of Engineering and Built Environment, Universiti Kebangsaan Malaysia (UKM). The fully automated wind tunnel is named Pangkor after an island in Perak, Malaysia. Different measurement techniques are used to understand and validate the flow quality of the turbulent boundary-layer profiles at different locations and directions (wall-normal and spanwise). A hot-wire sensor captures the boundary layer over a smooth, flat surface. These data are then compared with high-quality published data. The flow uniformity shows comparable velocity variations and turbulent intensity. The boundary-layer profiles collapse well in different spanwise locations. Furthermore, the boundary-layer profiles at different
Keywords
- wind tunnel
- turbulent boundary layer
- turbulent flow
- hot-wire anemometer
- boundary layer experiments
1. Introduction
Wind tunnels have been essential for aerodynamic research since the dawn of aviation [1]. Despite changes in their role and occasional predictions of their obsolescence, this situation is unlikely to change in the foreseeable future. Therefore, up-to-date measurement techniques are worthwhile. Constructing a new low-speed wind tunnel to study the fundamentals of turbulent boundary layers is a challenging endeavor that requires a profound comprehension of boundary layer dynamics and wind tunnel design [2]. These tunnels must undergo thorough testing to simulate typical boundary layer profiles with minimal turbulence intensity accurately. Extended wind tunnel studies are essential for advancing novel aircraft, wind turbines, and other systems that delicately interact with airflow. Low-speed aerodynamics is gaining popularity in both industry and academics. Despite the progress made in numerical models, low-speed wind tunnels remain essential in research and design procedures due to their unparalleled capacity to offer precise real-world data [3]. A smooth, turbulent boundary layer is necessary for sensitive experiments, where a high-quality flow becomes increasingly important [4]. One of the main variables affecting flow in a wind tunnel is the severity of turbulence. During the wind tunnel design process, much work is put into ensuring that the test section flow may have low levels of turbulence and stability. The main methods employed in the flow straightening and turbulence reduction system are contractions, honeycomb, and wire-mesh screens [5]. Most studies agreed that the screens and contractions reduce turbulence and mean velocity variation more in the longitudinal than in the lateral direction [3]. In the present research, a new low-speed wind tunnel was built in the Mechanical and Manufacturing Engineering Department, Universiti Kebangsaan Malaysia. This study aims to provide different measurement techniques using hot-wire anemometer. This method would help understand the turbulent boundary layer flow in wind tunnels.
2. Methodology
This study intends to systematically evaluate and validate the turbulent boundary layer profiles in a newly built wind tunnel. An extensive amount of data was gathered by employing various measuring approaches, each offering a unique set of benefits. A complete comparison and validation of the characteristics of the turbulent boundary layer were carried out by comparing the current data to high-quality published data [6, 7]. This was accomplished by employing sophisticated analytical tools to evaluate the data. A more reliable and in-depth understanding of turbulent boundary layers is vital for advancing wind tunnel and fluid mechanics.
2.1 Wind tunnel layout
Despite being owned by the Mechanical and Manufacturing Engineering Department, the Pangkor low-speed wind tunnel (PLSWT) is located at the Coastal and Water Resources Laboratory of the Faculty of Engineering and Built Environment, Universiti Kebangsaan Malaysia (UKM). The laboratory belongs to the Department of Civil Engineering, where research on waves, hydraulics using a wave maker, and channel flows are conducted. The wind tunnel has been developed and fine-tuned for the current research to improve flow quality and the turbulent boundary layer pattern. The wind tunnel is powered by a 15 kW AC axial fan, followed by a flexible duct, transition duct, diffuser, settling chamber, contraction nozzle with an area ratio of 2.4:1 and a test section with cross-sectional area 1.2 × 0.476 m (width × height) and 3 m total length. Figure 1 shows a general outline of the wind tunnel’s main parts. Figure 2 shows the actual photo of the wind tunnel.

Figure 1.
General outline of Pangkor low-speed wind tunnel.

Figure 2.
Pangkor low-speed wind tunnel. The left photo shows the main parts of the wind tunnel, starting from the transition duct to the contraction nozzle. The second photo shows the test section, and the traverse system is on the top of the ceiling.
The design and construction of the PLSWT started in 2014. The wind tunnel operated for the first time in September 2015 with a turbulence intensity level of 5%. To start with, the wind tunnel had only minimal measures to address turbulence intensities, that is, two meshing screens at the diffuser section. However, this value needed to be more suitable for the researchers to perform experiments to study the fundamentals of the turbulent boundary layer. In 2017, the second stage started with combining honeycomb and meshing screens to fine-tune the turbulent boundary layer profile and flow quality. Four new layers of meshing screens and a low-cost honeycomb built in-house, a three-dimensional plywood frame with dimensions of height,

Figure 3.
The variation of the mean velocity profile (a) and turbulence intensity (b) over the spanwise direction (y) of the wind tunnel. The vertical red dashed line indicates the center of the test section in a vertical direction.
In early 2018, serious development and fine-tuning for the wind tunnel were achieved. A new floor made of solid wood has been installed. Also, three stages of tripwires were used to develop the boundary layer. Therefore, this converted the wind tunnel to be the first for turbulent boundary layer studies in Malaysia with a minimum velocity variation and turbulence intensity value. For further information about the list of wind tunnels in Malaysia, see a review paper by Wiriadidjaja et al. [10].
2.2 Traverse construction
The traversing mechanism regulates the motion required for the installed sensors, such as the Pitot tube, hot-wire, or temperature probes, in both the vertical and horizontal directions. This enables various motions that may cover many locations within the examined sample. A two-dimensional (2D) traverse system designed and manufactured in-house includes two sliders firmly positioned within two sets of guides. These guides can be positioned vertically or horizontally and sit above the ceiling of the test section. Two fine-stepper motors facilitate the propulsion for this traverse. Therefore, each revolution necessitates 800 steps. The Vecta Steppers motors, specifically the PK266-034 model, are connected to Velmex controllers, the VXM-3 model. These motors are fitted with ball screws with a pitch of 1.6 mm, which applies to vertical and horizontal movements. The traverse provides a level of precision of less than 0.1 mm, or 4 × 10−3 mm for each step. This precision is necessary to capture the velocity fluctuations especially in the near-wall region. The motor controllers provide good support because communication can be established with many programming languages.
2.3 Data acquisition and automation
The most essential features of the wind tunnel are its functionalities and ease of use. A single-point control is preferred because operations can be made simple. Scilab is the only software for controlling air flow speeds, traverse systems, and acquisition operations. Starting from the motor, a National Instrument (NI) data acquisition (DAQ) system sends signals to the variable frequency drives (VFD). The fan speed was controlled through an output voltage signal produced by the NI DAQ 6008 module. This procedure is essential for hot-wire calibration. The atmospheric conditions, for example, atmospheric pressure (p), temperature (T), and relative humidity (RH), were measured using the Comet H7331 device for all experiments. The device was connected to the PC
2.4 Calibration procedure
The hot-wire sensor is calibrated within the wind tunnel in all experimental series, employing a Pitot tube. The calibration process is conducted twice throughout each experiment, known as pre-calibration and post-calibration. To properly calibrate the hot-wire sensor, it is necessary to position it at a free-stream location, specifically at the center of the test section. Following that, it is essential to systematically measure and record a series of velocities, incrementally ranging from zero up to about 50% beyond the free-stream velocity deemed necessary for the experiment. Consequently, a polynomial third-order fitting is employed to establish a relationship between the voltage measured by the hot wire and the velocity measured by the Pitot tube. A comparison sample of calibration data is presented in Figure 4. This example includes both pre-calibration and post-calibration data. The pre-calibration and post-calibration curves are not expected to collapse because of expected temperature drift. A temperature drift of 1°C is usually acceptable.

Figure 4.
Hot-wire calibration curve for
Even though there is an increasing variation as velocity is increased as shown in Figure 4, the current comparison is deemed acceptable. When there is a large temperature drift that can be caused by the wind tunnel fan that keeps producing heat or simply because the building absorbs more energy as the sun rises, a temperature compensation procedure can be implemented. A temperature compensation is a procedure when an interpolation between pre- and post-calibration is performed. A typical acceptance criterion is the free-stream velocity should be within 2%.
2.5 Determining initial distance between wall and hot wire
A manual measurement established the distance between the hot-wire sensor and the wall. The distance was determined accurately by employing a high-resolution camera and a ruler. An exact traverse system was crucial for effectively controlling the exact movement of the hot wire in millimeter increments. Consequently, the hot-wire sensor was positioned successfully at approximately

Figure 5.
Determining the distance between the wall and hot wire using the physical method.
3. Results and discussion
3.1 Flow uniformity
In order to ensure that the wind tunnel has a high-quality flow condition, a spanwise measurement was performed to analyze the velocity and turbulence intensity variability profiles. The measurements were conducted at the center of the test section at a distance of wall-normal
3.2 Turbulent boundary layer variability
A further check on the flow condition was performed. However, the focus this time is not on the homogeneity of flow in the center of the test section but on evaluating the quality of the turbulent boundary layer profile in various spanwise directions (y), as indicated in Figure 6. This is done to guarantee that the wind tunnel’s boundary layer profile follows the standard pattern and does not suffer from any defect. Figure 6 shows the wind tunnel test section and the location of spanwise measurements. The solid red line indicates the center of the test section, denoted by the letter C. The dashed red lines show the spanwise location of the turbulent boundary layer measurements denoted by 20 L, 10 L, 10R, and 20R. The letters L and R indicate the left and right directions about the center. The meaning of 10 L is that the measurement point shifted 100 mm to the left direction from the center point (C), and 20 L is shifted 200 mm from the center point (C). The points 10R and 20R shifted in the right direction from the center point (C). In the current experiment, there are five measurement points, each point separated by 100 mm, thus a coverage of 400 mm.

Figure 6.
Schematic diagram of the turbulent boundary layer measurements in different spanwise locations (y). The solid red line indicates the center of the test section, denoted by the letter C.
The wind tunnel is set to a free-stream velocity of
3.2.1 Diagnostic plot
The diagnostic plot was introduced by Alfredsson et al. [17] and Alfredsson et al. [18] as a method for evaluating the quality of the turbulent boundary layer. One significant feature of the diagnostic plot is that it does not require knowing the wall position or the friction velocity, therefore ensuring the elimination of any confusion relating to the position of the wall and the velocity related to friction. In their investigation of a smooth-wall turbulent boundary layer subjected to a zero pressure gradient (ZPG) environment [17, 18, 19, 20], it has demonstrated that the turbulent intensity, represented by

Figure 7.
Diagnostic plot of smooth turbulent boundary layer variability. Symbols (
3.2.2 Turbulence statistics: velocities and turbulence intensity profiles
Given the lack of direct measurements of
Figure 8 shows the mean velocities profile comparison between five locations in the spanwise direction. The velocity scaled with the skin-friction velocity
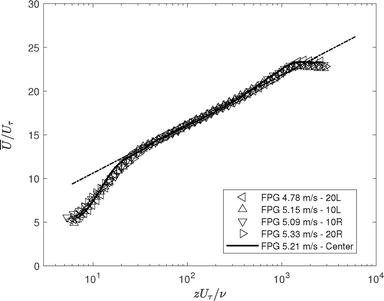
Figure 8.
Comparison of the mean velocity profiles of turbulent boundary layer of five points in the spanwise direction (y). The dashed-dot black line indicates the log law, here κ = 0.41 and a = 5.0. Symbols (
Figure 9 shows the velocity defect of all five profiles. The experiment data show an excellent agreement for the entire boundary layer. However, the current profiles do not follow the standard behavior of a turbulent boundary layer subjected to zero pressure gradient (ZPG). When the boundary layer is subjected to ZPG environment, the outer layer similarity profiles collapse well and agree with Townsend’s outer layer similarity [7, 24]. In other words, all the profiles in the ZPG case collapse well with the velocity defect low, represented by the following equation,

Figure 9.
Comparison of the velocity defect of five points in the spanwise direction. The dashed black line represents the defect low. Symbols (
where
Figure 10 compares the turbulent intensity profiles of all five locations. The inner peak follows the standard boundary layer profile at

Figure 10.
Comparison of the turbulent intensities of five points in the spanwise direction. Symbols (

Figure 11.
Comparison of the turbulent intensities of five points in the spanwise direction. Symbols (
4. Flow validation and smooth-wall case
An experiment of a wall-normal was performed over the smooth wall at three different free-stream velocities
Exp Code | Surface Type | Location | ||||
---|---|---|---|---|---|---|
S | Smooth | 50 | 5.22 | 87.3 | 0.2241 | 1204 |
S | Smooth | 50 | 8.01 | 115.2 | 0.3380 | 2395 |
S | Smooth | 50 | 10.27 | 127.8 | 0.4250 | 3410 |
Table 1.
4.1 Diagnostic plot
Figure 12 compares the diagnostic plot of the smooth surface and the smooth pressure gradient data taken from Harun [7]. The top figure shows the classical scaling of the diagnostic plot, where the turbulent intensity

Figure 12.
Comparison of diagnostic plot of the smooth data of the current experiments and FPG data taken from Harun [
4.2 Turbulence statistics: velocities and turbulence intensity profiles
Figure 13 shows the velocity and turbulent intensity profiles. The current comparison between all profiles of the current experiments is represented by the symbols (

Figure 13.
Comparison of mean velocity and turbulent intestines of the smooth data of the current experiments and FPG data taken from Harun [
Furthermore, Figure 14 shows the mean velocity profile (top) and turbulent intensity profiles (bottom) are scaled with the outer parameter. Where the velocity and turbulent intensity are normalized with free-stream velocity

Figure 14.
Comparison of mean velocity and turbulent intestines of the smooth data of the current experiments and FPG data taken from Harun [
Figure 15 compares the velocity defect between both experimental data. All the profiles show an excellent collapse for the entire boundary layer regions. All the profiles shifted downward due to the effect of the pressure gradient on the turbulent boundary layer [24, 28]. Overall, all the analyses provided for validating the smooth-wall case over the wind tunnel floor reveal that the turbulent boundary layer has high-quality data and follows the standard features for the boundary layer. All the differences between the current experiments and the referenced data taken from Harun [7] are within the acceptable limit.

Figure 15.
Comparison of velocity defect profile of the smooth data of the current experiments and FPG data taken from Ref. [
4.3 Spectral analysis
A spectral analysis was conducted to evaluate and understand the distribution and significance of various scale features in the turbulent boundary layer in both the near-wall and outer regions. One way to illustrate this is by analyzing all possible length scales that can exist at a particular location with respect to the wall. A pre-multiplied streamwise energy spectra provide a quick way to understand this, as the length scale can be non-dismensionalized with the boundary layer thickness (
The streamwise length scale is presented by

Figure 16.
Individual energy spectral at selected location. (a) z+ = 15 in the near-wall region, and (b)
On the other hand, Figure 16(b) shows that the highest energy of the flow structure at the outer region of

Figure 17.
Spectra maps for the flow over wind tunnel smooth surface (a) 5 m/s, (b) 8 m/s, and (c) 10 m/s. Symbols (+) and (×) represent the near-wall and outer hump locations.
5. Conclusions
Flow quality and turbulent boundary layer experiments have been performed at the Pangkor low-speed wind tunnel (PLSWT). The measurements were carried out using a hot-wire sensor over a flat surface. Different measurement techniques were used to understand and validate the flow in the wind tunnel’s test section. The flow uniformity measurement shows excellent results, where the wind tunnel experiences very low-level velocity and turbulent intensity variation due to the effects of the meshing screen and honeycomb device. The turbulence statistical analysis, including diagnostic test, boundary layer velocity profile, outer layer similarity, and turbulent intensity of different spanwise locations, shows a very excellent collapse between all five locations; this indicates that the boundary layer profiles across the spanwise direction do not differ and not suffer from any defect. The analysis of the turbulent boundary layer at the center of the test section at different
Acknowledgments
We would like to express our gratitude for the financial support provided by Universiti Kebangsaan Malaysia’s Research University Grant GUP-2020-015.
References
- 1.
Baals DD. Wind Tunnels of NASA. Vol. 440. Scientific and Technical Information Branch, National Aeronautics and Space …; 1981 - 2.
de Almeida O, Carnevalli F, de Miranda O, Neto F, Saad FG. Low subsonic wind tunnel-design and construction. Journal of Aerospace Technology and Management. 2018; 10 - 3.
Britcher C, Landman D. Wind Tunnel Test Techniques: Design and Use at Low and High Speeds with Statistical Engineering Applications. Academic Press; 2023 - 4.
Scheiman J, Brooks JD. Comparison of experimental and theoretical turbulence reduction from screens, honeycomb, and honeycomb-screen combinations. Journal of Aircraft. 1981; 18 (8):638-643 - 5.
Harun Z, Wan AW, Ghopa SA, Izhar Ghazali M, Abbas AA, Rasani MR, et al. The development of a multi-purpose wind tunnel. Jurnal Teknologi. 2016; 78 :6-10 - 6.
Harun Z, Monty JP, Mathis R, Marusic I. Pressure gradient effects on the large-scale structure of turbulent boundary layers. Journal of Fluid Mechanics. 2013; 715 :477-498 - 7.
Harun Z. The structure of adverse and favourable pressure gradient turbulent boundary layers [PhD thesis]. 2012 - 8.
Cattafesta L, Bahr C, Mathew J. Fundamentals of wind-tunnel design. In: Encyclopedia of Aerospace Engineering. Wiley Online Library; 2010. pp. 1-10 - 9.
Mathew J, Bahr C, Carroll B, Sheplak M, Cattafesta L. Design, fabrication, and characterization of an anechoic wind tunnel facility. In: 11th AIAA/CEAS Aeroacoustics Conference. Monterey, California: AIAA Aerospace Research Central; 2005. p. 3052 - 10.
Wiriadidjaja S, Hasim F, Mansor S, Asrar W, Rafie ASM, Abdullah EJ. Subsonic wind tunnels in Malaysia: A review. Applied Mechanics and Materials. 2012; 225 :566-571 - 11.
Harun Z, Abbas AA, Mohammed Dheyaa R, Ghazali MI. Ordered roughness effects on NACA 0026 airfoil. IOP Conference Series: Materials Science and Engineering. 2016; 152 :012005 - 12.
Hutchins N, Choi K-S. Accurate measurements of local skin friction coefficient using hot-wire anemometry. Progress in Aerospace Sciences. 2002; 38 (4-5):421-446 - 13.
Monty JP, Harun Z, Marusic I. A parametric study of adverse pressure gradient turbulent boundary layers. International Journal of Heat and Fluid Flow. 2011; 32 (3):575-585 - 14.
Harun Z, Abbas AA, Lotfy ER, Khashehchi M. Turbulent structure effects due to ordered surface roughness. Alexandria Engineering Journal. 2020; 59 (6):4301-4314 - 15.
Hutchins N, Marusic I. Evidence of very long meandering features in the logarithmic region of turbulent boundary layers. Journal of Fluid Mechanics. 2007; 579 :1-28 - 16.
Monty JP, Hutchins N, Ng HCH, Marusic I, Chong MS. A comparison of turbulent pipe, channel and boundary layer flows. Journal of Fluid Mechanics. 2009; 632 :431-442 - 17.
Henrik Alfredsson P, Örlü R. The diagnostic plot—A litmus test for wall bounded turbulence data. European Journal of Mechanics-B/Fluids. 2010; 29 (6):403-406 - 18.
Henrik Alfredsson P, Segalini A, Örlü R. A new scaling for the streamwise turbulence intensity in wall-bounded turbulent flows and what it tells us about the “outer” peak. Physics of Fluids. 2011; 23 (4). Article Number: 041702 - 19.
Henrik Alfredsson P, Örlü R, Segalini A. A new formulation for the streamwise turbulence intensity distribution in wall-bounded turbulent flows. European Journal of Mechanics-B/Fluids. 2012; 36 :167-175 - 20.
Henrik Alfredsson P, Segalini A, Örlü R. The diagnostic plot—A tutorial with a ten year perspective. In: iTi Conference on Turbulence. Springer; 2021. pp. 125-135 - 21.
Clauser FH. Turbulent boundary layers in adverse pressure gradients. Journal of the Aeronautical Sciences. 1954; 21 (2):91-108 - 22.
Clauser FH. The turbulent boundary layer. Advances in Applied Mechanics. 1956; 4 :1-51 - 23.
Nagib HM, Chauhan KA. Variations of von Kármán coefficient in canonical flows. Physics of Fluids. 2008; 20 (10). Article Number: 101518 - 24.
Townsend AAR. The Structure of Turbulent Shear Flow. Cambridge University Press; 1976 - 25.
Dróżdż A, Elsner W, Drobniak S. Scaling of streamwise Reynolds stress for turbulent boundary layers with pressure gradient. European Journal of Mechanics-B/Fluids. 2015; 49 :137-145 - 26.
Hutchins N, Marusic I. Large-scale influences in near-wall turbulence. Philosophical Transactions of the Royal Society A: Mathematical, Physical and Engineering Sciences. 2007; 365 (1852):647-664 - 27.
Harun Z, Isa MD, Rasani MR, Abdullah S. The effects of spatial resolution in turbulent boundary layers with pressure gradients. Applied Mechanics and Materials. 2012; 225 :109-117 - 28.
Townsend AA. The Structure of Turbulent Shear Flow. Cambridge: Cambridge University Press; 1956